Effect of pyridoxine in attenuating pulmonary pyroptosis-induced by third-hand smoke exposure: An in silico and in vivo study in male Wistar rats
Abstract
Third-hand smoke (THS) refers to the residual toxic substances that settle on surfaces following the combustion of tobacco. Although concerns about its health implications are increasing, studies exploring its impact on the lungs and potential protective strategies remain scarce. Thus, this study explored the potential of pyridoxine (vitamin B6), known for its anti-inflammatory and antioxidant effects, to alleviate THS-induced pulmonary pyroptosis, using both in silico modelling and in vivo experimentation. Molecular docking was employed to evaluate the binding affinity of pyridoxine to key molecular targets involved in pyroptosis, followed by a 30-day in vivo investigation using 30 male Wistar rats (n = 6 per group). The animals were randomly assigned to five groups: a control group exposed to clean air, and four groups subjected to THS exposure equivalent to 12 cigarettes/day. Of the THS-exposed groups, three received oral pyridoxine (3.5, 7, and 14 mg/kg body weight). On day 30, several parameters were assessed: cotinine levels (blood and urine), pyridoxine concentrations (lung and blood), lung pyroptosis markers (Caspase-1 activity, cleaved Gasdermin D, Interleukin-18), and lung injury indicators (Interleukin-1β levels and histopathological alterations). Docking analysis revealed that pyridoxine engages the same binding site as Ac-FLTD-CMK, a well-characterised Caspase-1 inhibitor. In THS-exposed rats, pyridoxine administration resulted in dose-dependent reduction in Caspase-1 activity, cleaved Gasdermin D, and Interleukin-18 levels, alongside improvements in lung injury scores and associated markers. Collectively, these findings suggest that pyridoxine may confer a protective effect against THS-induced pulmonary pyroptosis.
INTRODUCTION
Pyridoxine, a water-soluble B vitamin, functions as a coenzyme in numerous metabolic pathways and is increasingly recognized for its antioxidant and anti-inflammatory roles, including modulation of cytokine expression and antibody production [1]. Deficiency in pyridoxine has been linked to increased susceptibility to inflammatory disorders, while supplementation has been shown to ameliorate such effects [2–4]. Notably, individuals with elevated inflammatory markers frequently present with reduced circulating pyridoxine levels, a pattern associated with various chronic inflammatory conditions [5].
Recent focus has turned to pyroptosis, an inflammatory form of programmed cell death characterized by caspase activation and the release of pro-inflammatory intracellular contents. While pyroptosis is acknowledged as a “double-edged sword” in tumour pathophysiology [6], it also plays a significant role in non-neoplastic diseases, particularly those affecting the lungs. It has been implicated in the pathogenesis of severe asthma [7, 8], fibrotic lung conditions such as asbestosis and silicosis, acute respiratory distress syndrome (ARDS) [9], and, more recently, COVID-19-associated pneumonia [10].
Simultaneously, the adverse effects of third-hand smoke (THS) exposure have drawn increasing attention. THS, defined as residual tobacco pollutants that persist on surfaces and in dust, has been shown to aggravate asthma via mast cell activation [11] and to induce leucocyte adhesion in lung tissue [12], indicating a pro-inflammatory influence through yet-undetermined cellular mechanisms.
Given the dual relevance of pyridoxine’s anti-inflammatory properties and the involvement of pyroptosis in lung pathology, investigating whether pyridoxine can mitigate THS-induced pyroptosis is of particular interest. However, no studies to date have examined this association. The present study aims to address this gap by evaluating the anti-pyroptotic potential of pyridoxine through both in silico and in vivo approaches in a THS-exposed rat model.
MATERIALS AND METHODS
Materials
One of Indonesia’s commercial unfiltered cigarettes, with the highest nicotine content available (2.5 mg/cigarette), was used in this study. A custom-built smoking apparatus, designed to simulate active smoking, generated tobacco smoke with a suction power of 3.6 kPa, regulated via a timer. The smoke was introduced into four transparent container cages (484 × 322 × 262 mm) that would later house the rats (Figure 1).
Chromatography-grade water (H₂O) and methanol (MeOH) were procured from Fisher Scientific, Fair Lawn, NJ, USA. The mouse/rat cotinine enzyme-linked immunosorbent assay (ELISA) kit was obtained from Calbiotech® (CA, USA), while ELISA kits for caspase-1 (CASP-1) activity, Interleukin-1β (IL-1β), and IL-18 were sourced from Elabscience® (China). Pyridoxine (pyridoxine hydrochloride) was purchased from Solarbio (China), and a general vitamin B6 ELISA kit was acquired from Abclonal, ZellBio (Germany). The cleaved Gasdermin D (Rat N-terminal GSDMD or cGSDMD) ELISA kit was obtained from Sunlong Biotech (China).
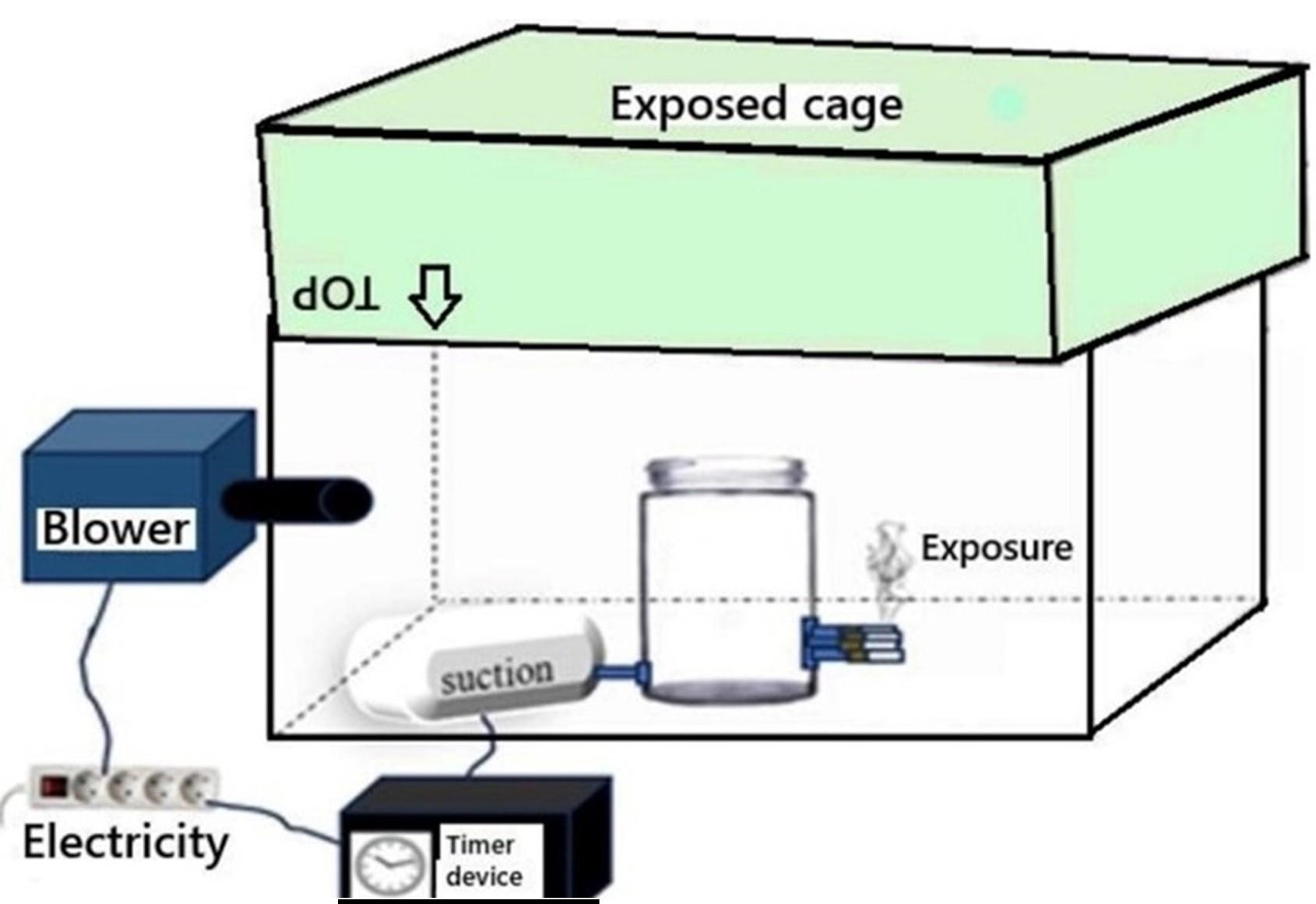
Container cages as the THS-environment model
Five transparent cages (484 × 322 × 262 mm) were assigned to five groups (Table 1). The four experimental cages were converted into THS-exposure environments using the smoking apparatus. The exposure process was conducted under controlled temperature conditions (24–25 °C) with no direct sunlight. To prevent degradation of nicotine residues, the cages were stored in a dark room for two days after each five-day exposure cycle. Following this period, the inner surfaces of all cages (including the control) were rinsed with ~25 mL of MeOH, collected in amber tubes, and sealed with Polytetrafluoroethylene (PTFE) tape to prevent leakage of a nicotine-containing solution. Gas chromatography-mass spectrometry (GC-MS) was performed to verify the presence of cigarette residues. This series of experiments was repeated weekly over 30 days (therefore, there were 3 cage replacement cycles), ensuring consistency in exposure conditions. The GC-MS test was performed at the Organic Chemistry Laboratory of the Faculty of Mathematics and Natural Sciences, Gadjah Mada University.
Table 1. The number of study groups and the implementation of exposure administration.
TSH rat model
Thirty male Wistar rats, aged seven weeks and weighing approximately 180 grams, were obtained from a local breeder in Yogyakarta, Indonesia. The rats were housed in the animal laboratory of the Anatomy Department, Faculty of Medicine, Gadjah Mada University, under controlled conditions with ad libitum access to water and food. The animals were randomly allocated into groups as described in Table 1. Rats exposed to THS were classified as “Third-Hand Smoker rats” based on the detection of elevated cotinine levels in their urine and/or blood compared to the control group.
This study adhered to institutional guidelines and received ethical approval from the Institutional Animal Care and Use Committee, Faculty of Medicine, Universitas Sebelas Maret Surakarta, Indonesia (Ethical Clearance No. 112/UN27.06.11/KEP/EC/2024).
Urine sampling, blood sampling, and tissue preparations
Urine samples were collected on day 30 of exposure, corresponding to the final day of THS exposure and seven days after the last weekly cage replacement (cage replacement 3). Each rat underwent 24-hour urine collection using metabolic cages. The collected urine samples were stored in clean tubes at -20°C until analysis. On day 31, the rats were sacrificed using a combination of ketamine, acepromazine, and xylazine. The lungs were resected and prepared for analysis. The left lung was preserved in 10% neutral buffered formalin (NBF) and stored at room temperature for histopathological examination. The right lung lobes were placed in a 1.5 mL microtube and stored at -80°C for molecular analysis. Blood samples were obtained on day 30 from the retro-orbital sinus and collected into 1.5 mL microtubes. The blood was centrifuged at 3000 rpm for 10 minutes, and the supernatant was transferred to a new 1.5 mL microtube and stored at -20°C until testing. Also, for a test using an automated blood analyser, 0.5 mL of blood was collected into Vacutainer® EDTA tubes. The sample was subsequently subjected to haematological analysis, including red blood cell count (RBC), white blood cell count (WBC), haemoglobin (Hb), and haematocrit (HCT).
Blood and urine
The ELISA assay was conducted to measure cotinine levels in blood and urine, as well as pyridoxine levels in blood and lung tissue. Lung samples were analyzed for cGSDMD, IL-1β, and IL-18 following the respective reagent protocols. Quantification using a colorimetric assay kit and a spectrophotometer was performed to measure lung CASP-1 activity, in accordance with the manufacturer’s instructions.
HE and MT staining of the rat’s lung
For histological assessment, the left lungs were collected and fixed in 10% buffered formalin. The tissues were embedded in paraffin blocks, sectioned into 5-µm-thick slices, and stained with haematoxylin and eosin (HE) as well as Masson’s trichrome (MT) staining. The prepared slides were examined under a light microscope at ×10 and ×40 magnification to assess structural changes, inflammatory infiltration, and possible fibrosis.
Molecular docking analysis
The structures of the target proteins were obtained from the RCSB Protein Data Bank (PDB) database, specifically Caspase-1 (PDB ID: 6BZ9). The protein structures were prepared by removing co-ligands, non-essential protein chains, and water molecules. Hydrogen atoms and charges were added using AutoDock Tools version 1.5.7 (Scripps Research, USA). For validation, redocking was performed using the native ligand from the crystal structures, and the root-mean-square deviation (RMSD) was calculated, with values ≤ 2.00 Å considered acceptable. The three-dimensional (3D) structure of pyridoxine (PubChem CID: 1054) was retrieved from the NCBI PubChem database, while Ac-FLTD-CMK was obtained from its respective protein structure in the PDB database. The ligands were prepared by adding hydrogen atoms and charges, and all rotatable bonds were set to be flexible using AutoDock Tools. Molecular docking was performed using AutoDock Vina, and the results were visualised using Biovia Discovery Studio Visualiser 2024 (Biovia, USA).
Statistical analysis
The data were analyzed using SPSS Statistics for Windows, Version 26.0 (IBM Corp., Armonk, NY, USA) and are presented as mean ± standard deviation (SD). The normality of the data distribution was assessed using Shapiro-Wilk test. For data that were not normally distributed, the Kruskal-Wallis test was employed, while one-way analysis of variance (ANOVA) was used for normally distributed data. Statistically significant ANOVA results were continued with post hoc analysis (Bonferroni). Statistical significance was set at p < 0.05. Correlation analyses were performed using either Spearman’s rank-order correlation or Pearson’s correlation coefficient, depending on the distribution of the data.
RESULTS
The cigarette-exposed cage: a " THS environment"
Gas Chromatography-Mass Spectrometry (GC-MS) analysis of the MeOH solution obtained from swabbing the inner surface of the cages detected cigarette residues in the exposed cages.
Compounds such as nicotine (pyridine), guaiene, and benzaldehyde were present in the exposed cages but absent in the control cages (Figure 2). Based on these findings, we considered the number of cigarettes, exposure duration, and dark-room storage period in this study sufficient to create a THS environment within the cages. Both exposed and control cages were used to house the experimental rats for 30 days, with cages being replaced weekly following the same procedure.
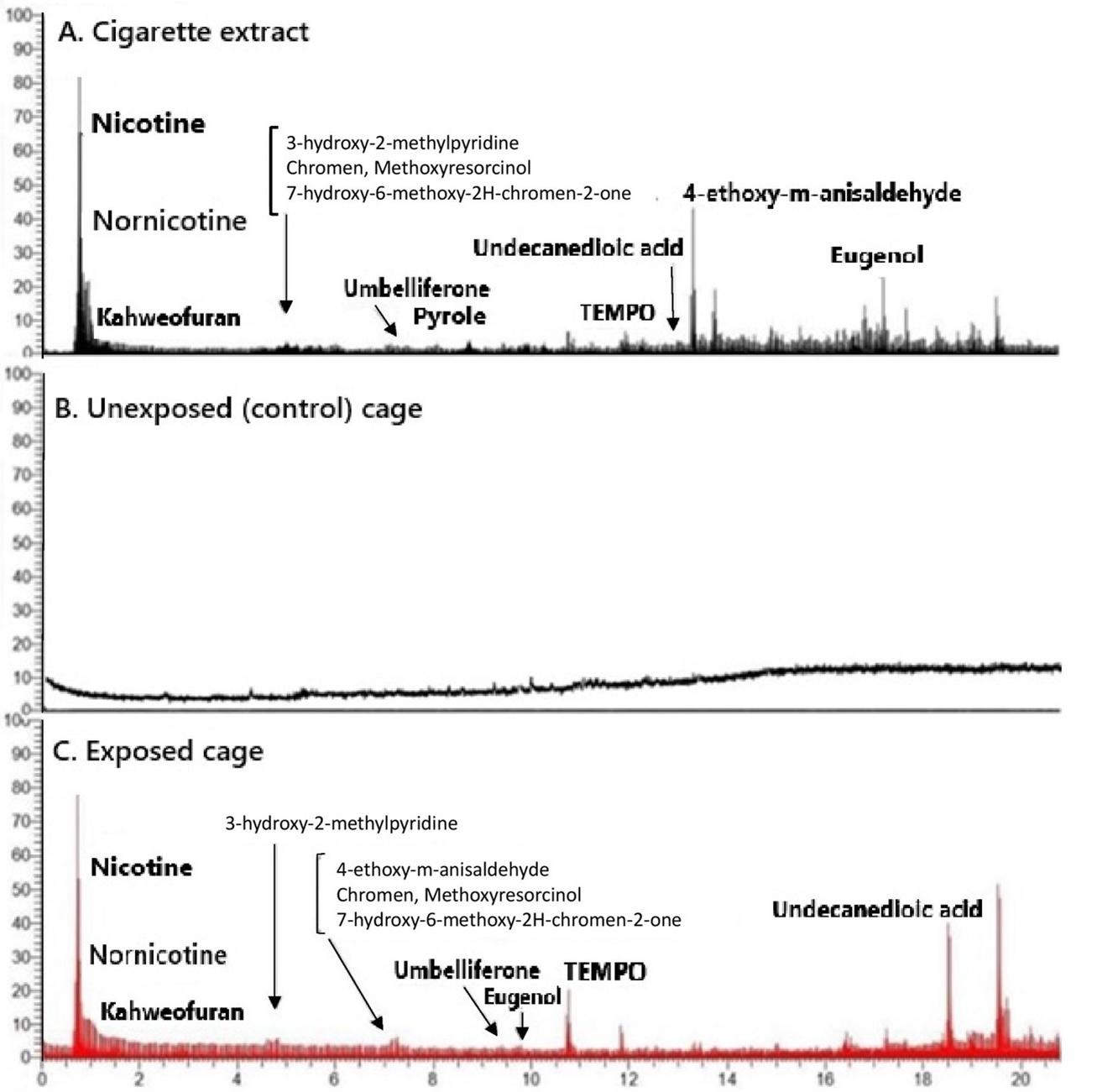
In silico analysis of pyridoxine shows a binding pocket that is similar to anti-CASP-1
Biocomputational analysis was carried out to find a potential protective effect of pyridoxine in decreasing the activity of inflammatory mediators that initiate pyroptosis: CASP-1. The 3D structures of both pyridoxine and CASP-1 were evaluated. Molecular docking results demonstrated that pyridoxine binds to these pyroptosis mediators, exhibiting a binding pocket similar to that of its respective antagonists (Table 2).
Evidence of a THS environment cage led to the next question: whether the cigarette residue adhered to the cage's inner surface could transfer to the rats’ bodies. To address this, the levels of nicotine metabolites, specifically cotinine, in the blood and urine across all groups were compared. Urine cotinine levels in THS-exposed rats were significantly higher than those in the control group (58.79 ± 36.05 vs 2.53 ± 0.65; Kruskal-Wallis p < 0.05). This is a confirmation that the THS-exposed rats had become a “third-hand smoker” rat model, as the increased nicotine metabolites suggest the transfer of other toxic residues into the rats’ bodies.
Table 2. Binding affinity and interaction sites of pyridoxine and Ac-FLTD-CMK with CASP-1.
The highest dose of pyridoxine showed lower urine and blood cotinine levels in THS rats
In three THS-exposed rats supplemented with Pyridoxine, the mean urine cotinine levels were lower compared to the THS-only group; however, significant differences were only observed in the group receiving the highest dose of Pyridoxine (THS+VitB6_3). The urine cotinine levels in this group were nearly identical to those of the control group (Figure 3). A similar pattern was observed in the blood.
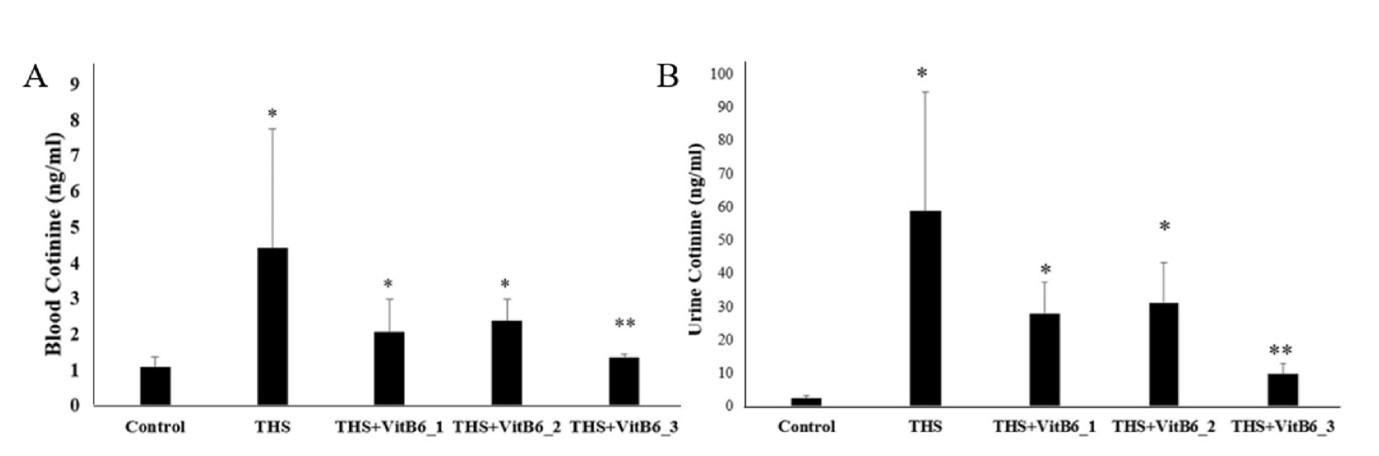
Supplementation of pyridoxine exhibited ameliorated lung pyroptosis in THS rats
Prior to evaluating the potential pulmonary effects of pyridoxine, this study first confirmed that rats housed in THS-exposed environments had indeed become valid “Third-Hand Smoker” models, as demonstrated in Figure 3. This verification prompted further investigation into whether THS exposure was sufficiently toxic to elicit pulmonary damage. In parallel, the pulmonary absorption of orally administered pyridoxine was assessed, with its presence in lung tissue confirmed (Figure 4D). Given that pyroptosis has been implicated in the pathogenesis of various lung disorders [7–10], an investigation into whether THS exposure could trigger such mechanisms was carried out.
Evidence of pyroptosis activation was reflected by elevated CASP-1 activity, and upregulation of cGSDMD and IL-18 levels in the THS group. The administration of pyridoxine was subsequently evaluated for its protective potential. Notably, pyridoxine supplementation significantly attenuated the expression of these pyroptosis-related markers in a dose-dependent manner. As illustrated in Figure 4A, the THS group exhibited the highest levels of CASP-1 activity, further confirming pyroptotic activation (4C, 4D), which were mitigated in the pyridoxine-treated groups.
Pyridoxine supplementation demonstrated a protective effect, as evidenced by lower levels of these markers in the supplemented groups. The higher the dose of pyridoxine, the lower the CASP-1 activity (Kruskal-Wallis p < 0.05), cGSDMD (ANOVA p < 0.05, with Games-Howell post hoc), and IL-18 (ANOVA p < 0.05, with Bonferroni), with all decreases being statistically significant. Detailed data for CASP-1 activity were as follows: 15.07 ± 4.37 (control) vs. 33.3 ± 5.47 (THS), 19.82 ± 5.24 (THS+VitB6_1), 17.47 ± 3.94 (THS+VitB6_2), and 16.37 ± 2.84 (THS+VitB6_3). For cGSDMD, the ELISA results were: 80.03 ± 6.21 (control) vs. 85.27 ± 1.2 (THS), 77.3 ± 4.57 (THS+VitB6_1), 77.58 ± 7.58 (THS+VitB6_2), and 73.94 ± 10.83 (THS+VitB6_3). For IL-18, the results were: 1954.48 ± 537.9 (control), 2877.3 ± 582.4 (THS), 1923.82 ± 547.5 (THS+VitB6_1), 1510.75 ± 490.4 (THS+VitB6_2), and 1431.17 ± 182.4 (THS+VitB6_3). The ELISA results for the lung injury marker (IL-1β) are as follows: 3684.4 ±1256.7 (control); 8324.5 ± 1610 (THS); 5967.3 ± 2279 (THS+VitB6_1); 3464.5 ± 2164.6 (THS+VitB6_2); 2279.3 ± 1350 (THS+VitB6_3). Correlation tests were also performed between pyridoxine supplementation and the various biomarkers. The correlation coefficient (r) values for IL-18 and cGSDMD were in the range of 0.4–0.59, indicating a moderate negative correlation (p < 0.05).
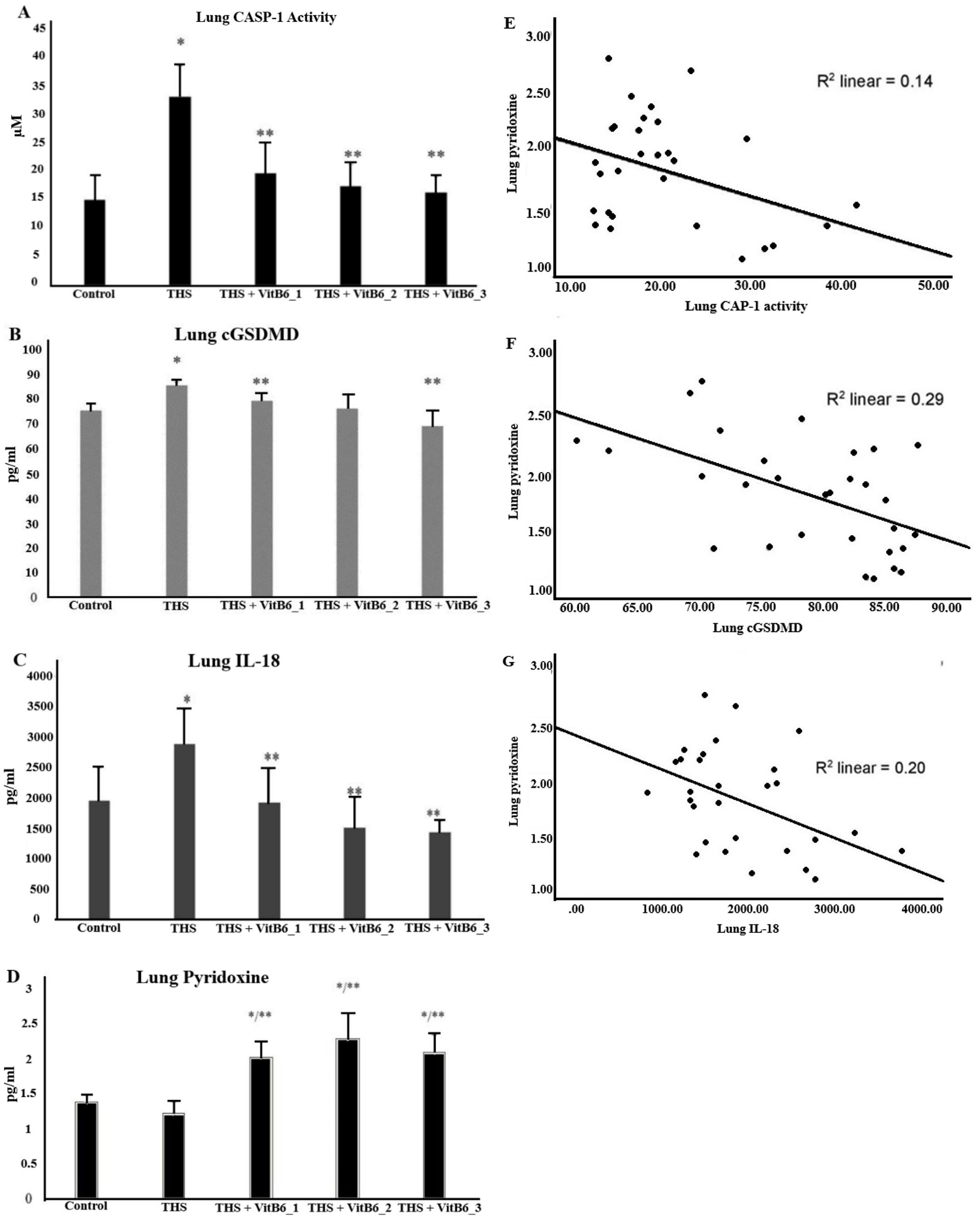
Supplementation of pyridoxine exhibited a reduced lung damage in THS rats
Exposure to THS consistently resulted in the detection of cigarette residues in the cages, increased cotinine levels in rat urine, and lung pyroptosis. The left lobe of the lungs was also examined using haematoxylin and eosin (HE) and Masson’s Trichrome (MT) staining. Compared to the control group, THS-exposed rats exhibited significant damage to the cellular structure of the lungs and small airways (Figure 5). In line with its effect on reducing inflammatory mediators associated with pyroptosis, pyridoxine supplementation also alleviated structural damage in the lungs and airways caused by THS exposure. Histological assessments with HE staining at magnifications of x10 and x40 included the examination of alveolar epithelium, inflammatory cell infiltration, protein debris, thickening of alveolar walls, and pneumocyte damage. As shown in Figure 5, rats in the THS group exhibited disordered epithelial cell structures in the terminal bronchioles, along with leucocyte infiltration between the alveoli.
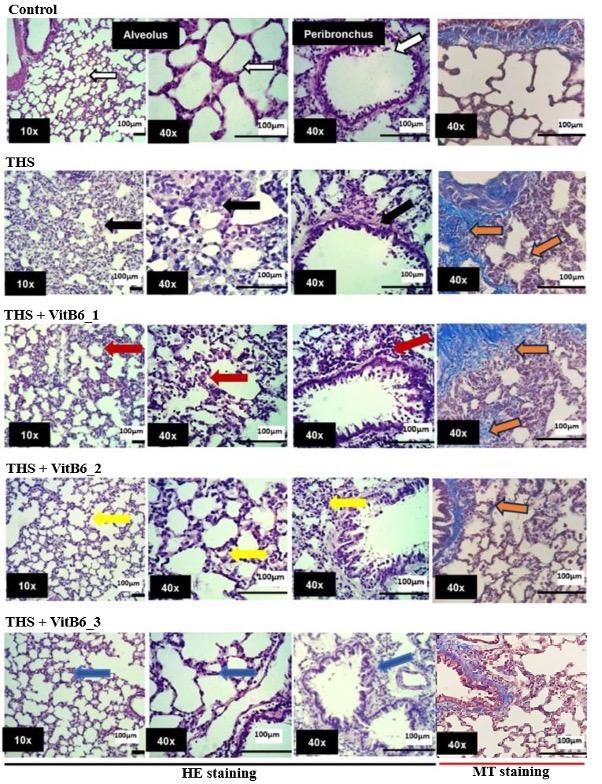
More than 50% of the visual field showed thickening of the alveolar walls, predominantly characterized by diffuse inflammatory cells. Peribronchial inflammatory cell infiltration was also apparent. Many alveoli exhibited single-layer pneumocyte walls, with increased cell infiltration in the alveolar septum. Protein debris was frequently observed, and several regions showed atelectasis. Using the scoring system developed by Pham et al. (2021) [13], which evaluated lung inflammation severity in Wistar Kyoto and Spontaneously Hypertensive rats following direct exposure to tobacco smoke, the control group in this study showed a lung inflammation severity score of 3.
In contrast, the overall structural damage in THS-exposed rats was significantly less in the pyridoxine-supplemented groups, particularly in terms of inflammatory cell infiltration.
Even the lowest dose of pyridoxine (VitB6_1) resulted in noticeably lighter structural damage compared to the THS group. As the dose increased, lung damage diminished, and normal lung structures were more frequently observed. A quantitative assessment was also conducted to provide supporting evidence of lung injury associated with pyroptosis occurring in the pulmonary tissue (Table 3; Figure 6A). The scoring systems were adapted from currently published literature within the past five years to ensure methodological relevance. Notably, the scoring approaches used were not intended to reflect acute lung injury (ALI), as the exposure in this study was administered over a period longer than one week. The assessment methods were adapted from Pham et al., 2021 [13] and Utari et al., 2018 [14]. THS exposure significantly increased lung injury scores compared to the control group. Treatment with Pyridoxine at the highest dose significantly reduced the injury scores relative to the THS group. The intermediate doses showed partial amelioration but did not reach statistical significance in all cases.
In addition, lung injury assessment was further supported by the measurement of IL-1β levels (Figure 6B), given that this cytokine is closely associated with the pyroptosis pathway [6,9,15-17], which underpins the central framework of the study. The results were consistent with other parameters, showing a significant increase in the THS-exposed group, while Pyridoxine administration effectively reduced IL-1β levels. Masson’s Trichrome (MT) staining was also performed to assess collagen deposition as an early indicator of potential fibrotic changes. The MT staining results revealed increased collagen deposition in THS group, whereas the THS + VitB6_2 and THS + VitB6_3 groups exhibited noticeably reduced levels.
Table 3. Lung injury scores in different treatment groups.
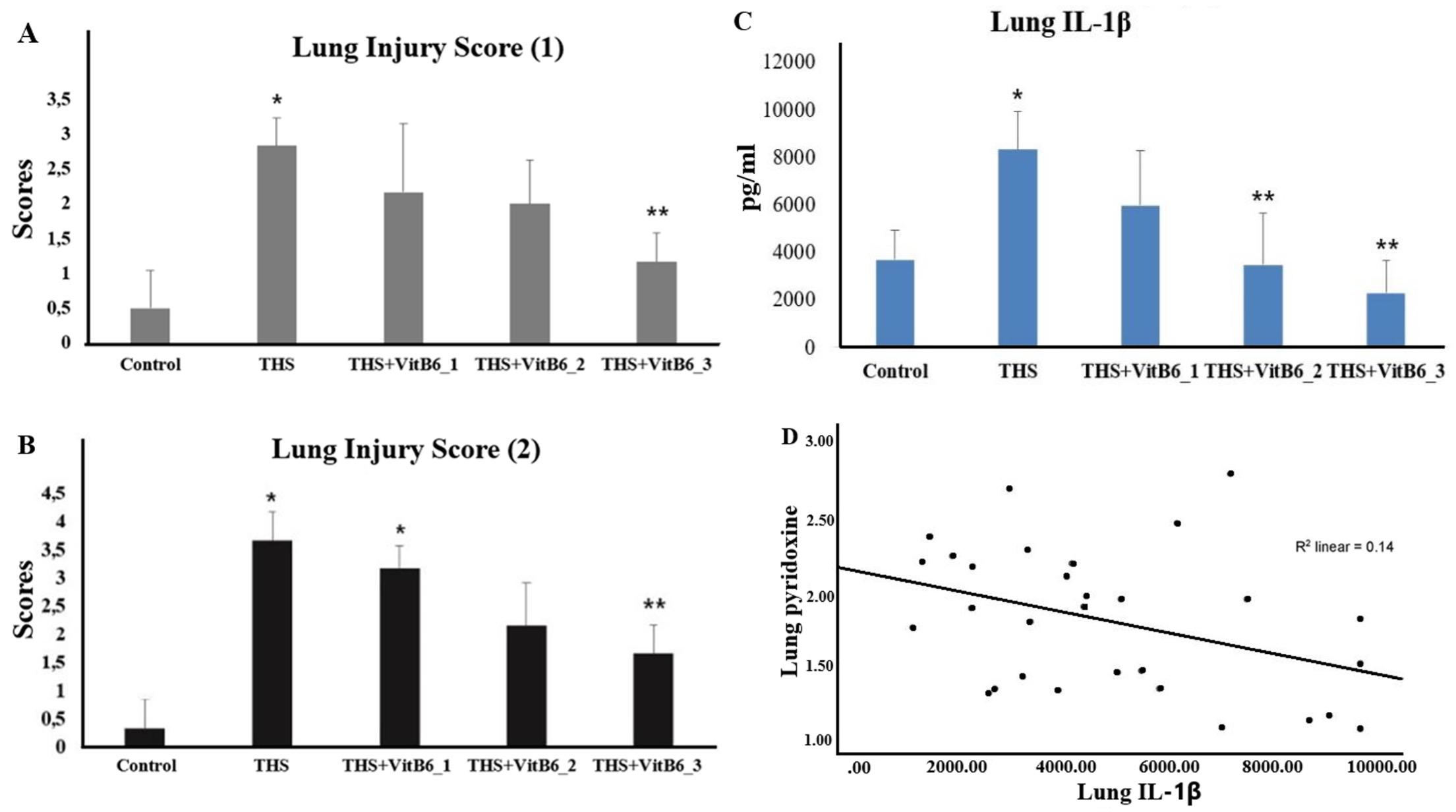
Pyridoxine mitigates haematological changes in THS rats
After 30 days of exposure, a blood examination focusing on haematological parameters reported in the literature to be influenced by cigarette smoke exposure [18] was carried out. All four parameters were significantly elevated in the THS-exposed group compared to the control, indicating that THS may also provoke haematological disturbances (Figure 7). Notably, pyridoxine supplementation in the exposed groups appeared to attenuate these effects, with haematological values shifting toward those observed in the control group (Table 4).
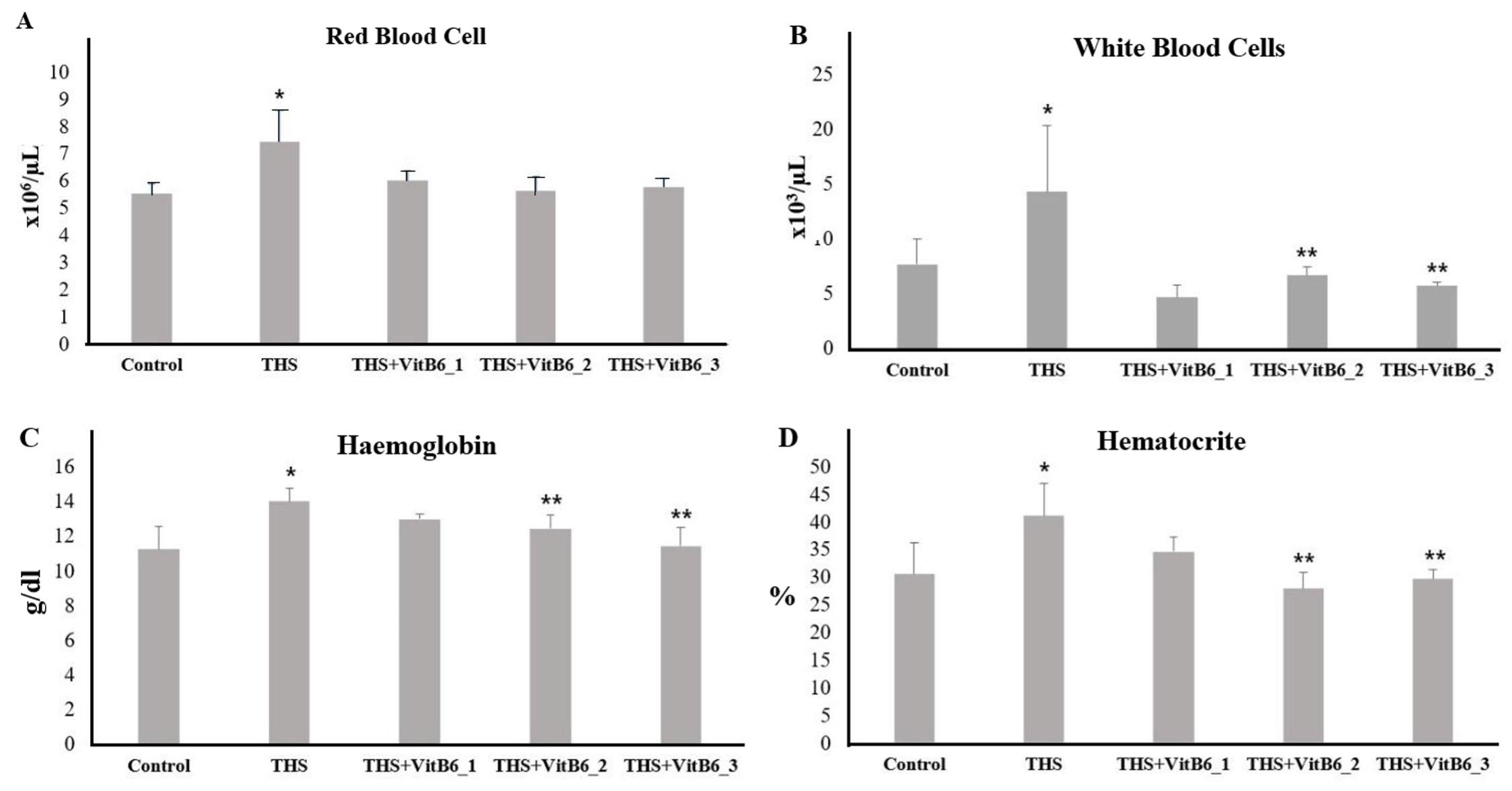
Table 4. Effects of THS and pyridoxine on haematological parameters in rats.
DISCUSSION
Third-hand smoke consists of residual compounds from cigarettes that persist in the environment, such as on surfaces, and may subsequently transform into toxicants. This poses a threat to non-smokers, as THS can be transferred and cause harm. Research on THS is essential, given that 22.3% of the world's population are active smokers [19], indicating that cigarette residues are an "everywhere-threat" for passive smokers. However, studies on THS remain limited, particularly regarding the potential protective effects of certain drugs or vitamins against this unavoidable exposure. Pyridoxine, commonly known as pyridoxine, has begun to attract attention for its anti-inflammatory properties [5,20-22]. Pyroptosis, an inflammatory-induced cell death mechanism, involves the activation of CASP-1 and is characterized by Gasdermin D cleavage, leading to pore formation, cell rupture, and IL-1β & IL-18 secretion. Pyroptosis is a major contributor to severe and difficult-to-treat lung diseases [9,10]. Despite their individual significance, the connection between THS exposure and the protective potential of pyridoxine against pyroptosis has not been studied. In this study, the protective effects of Pyridoxine against THS-induced pyroptosis in the lungs of the THS rat model were investigated. The exposure method consisted of a cycle of cigarette smoke fumigation (5 days of exposure with 12 cigarettes per day), followed by two days of storage in a dark room. The use of cigarettes with the highest nicotine content was intentional, as nicotine is a well-established primary constituent of cigarettes [23-29], serving as a marker residue and facilitating the detection of other toxic compounds. Cages were stored in a dark room to minimize the degradation of nicotine caused by exposure to sunlight [30]. Particular attention was given to this aspect solely for research purposes—specifically, to validate the THS environmental model. As will be discussed in the following paragraph, nicotine was selected as a THS marker in this study. By maximizing the likelihood of nicotine detection, the study could proceed toward its main objective: evaluating the effects of a specific vitamin, pyridoxine. The researchers considered that if there was no evidence of nicotine or other residue presence in the cage environment and/or no measurable increase in nicotine metabolites in the animals' urine, the process would be deemed insufficient. Therefore, focused attention was given to this aspect as part of an effort to strengthen the research's validity.
Chromatographic analysis confirmed the presence of cigarette residues in the exposed cages, establishing a "THS environment" for the rats. Figure 2A and 2C highlight the presence and transfer of volatile and semi-volatile compounds from cigarette smoke to the surrounding environment. Panel A (Cigarette extract) exhibits a diverse range of chemical constituents, with prominent peaks corresponding to nicotine, nornicotine, kahweofuran, and 4-ethoxy-m-anisaldehyde, alongside several other compounds such as eugenol, umbelliferone, and undecanedioic acid. These findings are consistent with known constituents of tobacco smoke, many of which possess toxicological relevance [31,32]. In contrast, panel B (Unexposed control cage) displays an absence of these compounds, confirming the baseline condition and validating that the control environment was free from contamination. This serves to underscore the fidelity of the experimental conditions and strengthens the comparative significance of the exposed samples. Panel C (Exposed cage) reveals a chromatographic profile that bears remarkable similarity to that of the cigarette extract, with identifiable peaks for key compounds such as nicotine, nornicotine,3-hydroxy-2-methylpyridine, eugenol, and TEMPO (2,2,6,6-tetramethylpiperidine 1-oxyl). The detection of these constituents in the cage environment following exposure indicates that volatile compounds from cigarette smoke are readily disseminated and adsorbed into the surrounding materials. The presence of shared peaks between the cigarette extract and the exposed cage sample strongly supports the hypothesis that environmental exposure to cigarette smoke results in passive contamination. In this study, nicotine, which is known for its environmental persistence and well-documented health risks, is considered a marker of THS exposure, in alignment with findings reported in existing literature [23-28].
To confirm whether the chemical residues present in the cage environment were transferred to the animals, cotinine levels in both urine and blood were measured. Cotinine, a well-established metabolite of nicotine, serves as a reliable biomarker for tobacco exposure [24, 33, 34]. The results in this study showed that THS-exposed rats exhibited significantly elevated cotinine levels compared to the control group. This finding provides evidence that chemical constituents from THS can be absorbed by living organisms, even in the absence of visible cigarette smoke. Despite the lack of active smoke at the time of exposure, the persistent residues deposited within the cage environment were sufficient to result in measurable internal exposure in the animals. This supports the premise that THS poses a latent but biologically significant exposure risk, reinforcing the importance of studying its health effects under controlled experimental conditions.
There has been limited research linking Pyridoxine to smoking status [29], but none have examined its relationship with nicotine levels from smoking. This study presents novel findings demonstrating that high doses of pyridoxine reduced cotinine levels in THS rats, bringing them close to control group levels. The cotinine reduction “appeared” dose-dependent, though statistical significance was only observed at the highest Pyridoxine dose. The observed variability in the results may be attributed to the inherent biological variability associated with in vivo studies, particularly when utilizing animal models. Although experimental conditions were carefully standardized, such fluctuations are not uncommon. Notably, the presence of an outlier within the THS+VitB6_2 group may have influenced the overall statistical outcomes. These findings are consistent with the known pharmacokinetic properties of nicotine and its primary metabolite, cotinine, particularly their respective half-lives. Given that cotinine exhibits a longer half-life (approximately 1–2 days) [24,26,29], urine samples offered a more stable and reliable matrix for detection compared to blood, thereby enhancing the sensitivity of exposure assessment [24]. Further investigation assessed whether pyroptosis (indicated by CASP-1 activity, cGSDMD, and IL-18), as well as lung injury (represented by IL-1β and lung injury score), were present after 30 days of THS exposure. Results confirmed pyroptosis and lung injury in the THS-exposed rats, with Pyridoxine supplementation significantly reducing its effects.
To understand pyridoxine's mechanism of action, molecular docking analysis was performed, revealing binding interactions between Pyridoxine and pyroptosis mediators. Pyridoxine showed hydrogen bonding at key sites on CASP-1 (His 237, Arg 179) with a binding affinity of -4.8 kcal/mol. Notably, pyridoxine's binding site was similar to the CASP-1 inhibitor Ac-FLTD-CMK, which prevents GSDMD cleavage and suppresses pyroptosis [35]. This suggests Pyridoxine may act as a non-competitive inhibitor, though further kinetic studies are necessary to confirm this hypothesis. The quantitative assessment of pyroptosis-associated markers supports this observation. This finding may provide an important clue that pyridoxine, identified in this study via in silico analysis as a potential inhibitor of CASP-1 activity, could exert its anti-pyroptotic effects at an earlier stage, specifically at the level of caspase activation, prior to GSDMD cleavage. Given that CASP-1 activation leads to GSDMD cleavage and IL-1β & IL-18 secretion, the reduction in these markers with Pyridoxine supplementation aligns with pyroptosis pathway mechanisms [15,16,21,35]. These findings also corroborate an in vitro study by Mikkelsen (2023) [20], which showed that high doses of Pyridoxine reduced CASP-1 gene expression. Histological examination of lung tissue using HE staining revealed pronounced structural damage in THS-exposed rats. Notable pathological features included disorganisation of the bronchiolar epithelium, infiltration of inflammatory cells, thickening of the alveolar walls, and the accumulation of proteinaceous debris. Quantitative analysis using a lung injury scoring system adapted from [13] and [14] further confirmed a significant increase in lung injury scores in the THS group compared to controls. These findings provide strong evidence that pyroptosis in the lungs induces substantial tissue injury, as reflected by the elevated injury scores. The evidence of injury was further supported by a significant increase in IL-1β levels observed in the THS group, consistent with the pro-inflammatory nature of pyroptotic cell death. Pyridoxine supplementation, particularly at high doses, mitigated these effects, preserving lung structure closer to normal.
Although the primary focus of this study is on pyroptosis, Masson's Trichrome (MT) staining was also performed to evaluate the presence of lung fibrosis. This approach was taken in consideration of the fact that sustained pyroptosis can drive chronic inflammation, which may subsequently lead to extracellular matrix accumulation and tissue remodelling [17,36]. While fibrosis was not the central aim of the investigation, the inflammatory responses associated with pyroptosis may contribute to early fibrotic alterations in lung tissue. Over the past five years, several studies have focused on the role of pyroptosis as an underlying mechanism in the development of fibrosis. Emerging evidence suggests that this process may be mediated, at least in part, by interleukin-18 (IL-18), which has been shown to activate pro-fibrotic signalling pathways [17,37-39].
This study’s findings support the notion that chronic or sustained pyroptotic activity not only contributes to inflammation but may also drive tissue remodeling and fibrotic progression. The MT staining revealed more prominent collagen accumulation in the peribronchial region, interalveolar septa, and interstitial areas in the THS group compared to the control. Returning to the primary objective of this study—to investigate the potential protective effects of Pyridoxine—it was observed that in rat groups supplemented with Pyridoxine, signs of fibrosis were still present, but appeared minimal in comparison with the THS-only group. Based on these fibrosis findings, it is hoped that the present results may serve as a valuable reference for future studies focusing more specifically on the protective potential of Pyridoxine against fibrosis. To date, this study proposes the assumption that Pyridoxine may reduce the development of fibrosis, potentially through the inhibition of pyroptosis-related pathways. It is important to reiterate that fibrosis is considered an irreversible condition. Therefore, based on the prevailing theoretical framework, the observation of reduced fibrosis in THS-exposed rats treated with Pyridoxine in this study is more appropriately interpreted not as a reversal of fibrosis, but rather as a consequence of the inhibition—or at least attenuation—of upstream processes, particularly pyroptosis [40].
The next parameter examined was haematology. The effect of THS exposure on haematological parameters, previously reported in cigarette smoke-related studies [18, 41], was also confirmed in the present investigation. Rats exposed to THS demonstrated significant alterations in Hb levels, HCT, RBC, and WBC counts compared to the control group. These findings indicate a systemic response to prolonged THS exposure, likely involving both erythropoietic stimulation and inflammatory processes [41,42]. Notably, supplementation with pyridoxine, particularly at higher doses, ameliorated these changes. In the THS+VitB6_2 and THS+VitB6_3 groups, WBC, Hb, and HCT values showed significant improvement, approaching those of the control group. This suggests a potential modulatory role of pyridoxine in counteracting haematological disturbances induced by THS, possibly through its anti-inflammatory and antioxidative properties. The findings from this study provide new insights into THS exposure, demonstrating a) THS exposure induces lung pyroptosis and lung injury; b) THS rats exhibit elevated cotinine levels that correlate with Pyridoxine supplementation; and c) pyridoxine protects against THS-induced lung pyroptosis and injury.
Previous studies have highlighted Pyridoxine's anti-inflammatory roles in sepsis [42], LPS-stimulated monocytes [20, 43], and pneumonia in mouse models [43]. This study extends these findings, proposing Pyridoxine as a potential anti-pyroptosis agent in THS exposure and possibly as a nicotine-reducing supplement.
CONCLUSIONS
This study demonstrates that pyridoxine supplementation exerts a protective effect against THS-induced lung pyroptosis by reducing CASP-1 activity, which consequently downregulates its downstream pathway components such as cGSDMD, IL-18, and lung injury, thereby suggesting its potential as a therapeutic intervention for mitigating the harmful effects of THS exposure. This study was conducted over 30 days, during which the group that did not receive vitamin supplementation exhibited pyroptosis. However, no comparative time points (e.g., 15 or 20 days) were assessed, leaving it unclear whether pyroptosis due to THS exposure might have occurred earlier. Additionally, this study included only male rats, so the potential influence of hormonal factors remains unknown. Another thing is that, as this study primarily focuses on molecular mechanisms, assessments closer to the clinical level, such as lung function tests, were not performed. We hope that this aspect may serve as a recommendation for future research to build upon and explore in subsequent studies.
ACKNOWLEDGEMENTS
Mr. Suratno: National Research and Innovation Agency, for his support in chromatography, Assoc-Professor Nur Arfian, Tiara Kurniasari, Ando Gavintri, and Safira: Anatomy Department, Faculty of Medicine, Gadjah Mada University, Indonesia for technical expertise in animal treatments. Fatin Asfarina: Laboratorium Riset Terpadu, Faculty of Medicine, Public Health and Nursing, Gadjah Mada University, Indonesia, for molecular laboratory assistance. Andika Prasetyo: Sebelas Maret University Surakarta, Indonesia, for his support in molecular docking.
AUTHOR CONTRIBUTIONS
APS carried out the conceptualization, methodology, experiments, analysis and was a major contributor in writing the manuscript. R, ADS, and DI participated in study design and coordination, as well as data curation and draft editing. All authors read and approved the final manuscript.
CONFLICTS OF INTEREST
There is no conflict of interest among the authors.
References
- [1]Benabdellah K, Azcón-Aguilar C, et al. GintPDX1 encodes a protein involved in vitamin B6 biosynthesis that is up-regulated by oxidative stress in the arbuscular mycorrhizal fungus Glomus intraradices. New Phytol. 2009;184(3):682–93.
- [2]Mikkelsen K, Stojanovska L, et al. The effects of vitamin B in depression. Curr Med Chem. 2016;23(38):4317–37.
- [3]Mikkelsen K, Stojanovska L, et al. The effects of vitamin B on the immune/cytokine network and their involvement in depression. Maturitas. 2017;96:58–71.
- [4]Mikkelsen K, Stojanovska L, Tangalakis K, Bosevski M, Apostolopoulos V. Cognitive decline: A vitamin B perspective. Maturitas. 2016;93:108–13.
- [5]Ueland PM, McCann A, et al. Inflammation, vitamin B6 and related pathways. Mol Aspects Med. 2017;53:10–27.
- [6]Yu P, Zhang X, et al. Pyroptosis: mechanisms and diseases. Signal Transduct Target Ther. 2021;6(1):128.
- [7]Sebag SC, Koval OM, et al. Mitochondrial CaMKII inhibition in airway epithelium protects against allergic asthma. JCI Insight. 2017;2(3):e88297.
- [8]Lachowicz-Scroggins ME, Dunican EM, et al. Extracellular DNA, neutrophil extracellular traps, and inflammasome activation in severe asthma. Am J Respir Crit Care Med. 2019;199(9):1076–85.
- [9]Feng Y, Li M, et al. Pyroptosis in inflammation-related respiratory disease. J Physiol Biochem. 2022;78(4):721–37.
- [10]Bittner ZA, Schrader M, et al. Pyroptosis and its role in SARS-CoV-2 infection. Cells. 2022;11(10):1717.
- [11]Yu M, Mukai K, et al. Thirdhand smoke component can exacerbate a mouse asthma model through mast cells. J Allergy Clin Immunol. 2018;142(5):1618–27.e9.
- [12]El-Mahdy MA, Abdelghany TM, et al. Chronic cigarette smoke exposure triggers a vicious cycle of leukocyte and endothelial-mediated oxidant stress that results in vascular dysfunction. Am J Physiol Heart Circ Physiol. 2020;319(1):51–65.
- [13]Pham AK, Wu CW, et al. Differential lung inflammation and injury with tobacco smoke exposure in Wistar Kyoto and spontaneously hypertensive rats. Inhal Toxicol. 2020;32(8):328–41.
- [14]Utari PD, Setroikromo R, et al. PvdQ quorum quenching acylase attenuates Pseudomonas aeruginosa virulence in a mouse model of pulmonary infection. Front Cell Infect Microbiol. 2018;8:119.
- [15]Jorgensen I, Miao EA. Pyroptotic cell death defends against intracellular pathogens. Immunol Rev. 2015;265(1):130–42.
- [16]Mathur A, Hayward JA, et al. Molecular mechanisms of inflammasome signaling. J Leukoc Biol. 2018;103(2):233–57.
- [17]Liu Y, Wang D, et al. Biological and pharmacological roles of pyroptosis in pulmonary inflammation and fibrosis: recent advances and future directions. Cell Commun Signal. 2024;22(1):586.
- [18]Hang B, Snijders AM, et al. Early exposure to thirdhand cigarette smoke affects body mass and the development of immunity in mice. Sci Rep. 2017;7:41915.
- [19]WHO (2023). Tobacco. World Health Organization. Available from: https://www.who.int/news-room/fact-sheets/detail/tobacco
- [20]Mikkelsen K, Dargahi N, et al. High-dose vitamin B6 (pyridoxine) displays strong anti-inflammatory properties in lipopolysaccharide-stimulated monocytes. Biomedicines. 2023;11(9):2578.
- [21]Zhang P, Tsuchiya K, et al. Vitamin B6 prevents IL-1β protein production by inhibiting NLRP3 inflammasome activation. J Biol Chem. 2016;291(47):24517–27.
- [22]Du X, Yang Y, et al. Vitamin B6 prevents excessive inflammation by reducing accumulation of sphingosine-1-phosphate in a sphingosine-1-phosphate lyase-dependent manner. J Cell Mol Med. 2020;24(22):13129–38.
- [23]Martins-Green M, Adhami N, et al. Cigarette smoke toxins deposited on surfaces: implications for human health. PLoS One. 2014;9(1):e86391.
- [24]Torres S, Merino C, et al. Biomarkers of exposure to secondhand and thirdhand tobacco smoke: recent advances and future perspectives. Int J Environ Res Public Health. 2018;15(12):2693.
- [25]Yeh K, Li L, et al. Thirdhand smoke from tobacco, e-cigarettes, cannabis, methamphetamine and cocaine: partitioning, reactive fate, and human exposure in indoor environments. Environ Int. 2022;160:107063.
- [26]Hang B, Wang P, et al. Adverse health effects of thirdhand smoke: from cell to animal models. Int J Mol Sci. 2017;18(5):932.
- [27]Matt GE, Quintana PJ, et al. Thirdhand tobacco smoke: emerging evidence and arguments for a multidisciplinary research agenda. Environ Health Perspect. 2011;119(9):1218–26.
- [28]Ferrante G, Simoni M, et al. Third-hand smoke exposure and health hazards in children. Monaldi Arch Chest Dis. 2013;79(1):38–43.
- [29]Ulvik A, Ebbing M, et al. Long- and short-term effects of tobacco smoking on circulating concentrations of B vitamins. Clin Chem. 2010;56(5):755–63.
- [30]Kandylioti I, Vione D, et al. Solar light photodegradation of nicotine in the presence of aged polystyrene microplastics. Sci Total Environ. 2024;919:170500.
- [31]Soleimani F, Dobaradaran S, et al. Content of toxic components of cigarette, cigarette smoke vs cigarette butts: a comprehensive systematic review. Sci Total Environ. 2022;813:152667.
- [32]Centers for Disease Control and Prevention. How tobacco smoke causes disease: the biology and behavioral basis for smoking-attributable disease. In: National Center for Chronic Disease Prevention and Health Promotion, Office on Smoking and Health (eds). CDC, Atlanta, GA, 2010. Available from: https://www.ncbi.nlm.nih.gov/books/NBK53014/
- [33]Benowitz NL, Jain S, et al. Urine cotinine screening detects nearly ubiquitous tobacco smoke exposure in urban adolescents. Nicotine Tob Res. 2017;19(9):1048–54.
- [34]Mourino N, Ruano-Raviña A, et al. Serum cotinine cut-points for secondhand smoke exposure assessment in children under 5 years: a systemic review. PLoS One. 2022;17(5):e0267319.
- [35]Yang J, Liu Z, et al. Mechanism of gasdermin D recognition by inflammatory caspases and their inhibition by a gasdermin D-derived peptide inhibitor. Proc Natl Acad Sci U S A. 2018;115(26):6792–7.
- [36]Song Z, Gong Q, Guo J. Pyroptosis: mechanisms and links with fibrosis. Cells. 2021;10(12):3509.
- [37]She YX, Yu QY, Tang XX. Role of interleukins in the pathogenesis of pulmonary fibrosis. Cell Death Discov. 2021;7(1):52.
- [38]Janho Dit Hreich S, Juhel T, et al. Activation of the P2RX7/IL-18 pathway in immune cells attenuates lung fibrosis. Elife. 2024;12:RP88138.
- [39]Demarco B, Danielli S, et al. How pyroptosis contributes to inflammation and fibroblast-macrophage cross-talk in rheumatoid arthritis. Cells. 2022;11(8):1307.
- [40]Antar SA, Ashour NA, et al. Fibrosis: types, effects, markers, mechanisms for disease progression, and its relation with oxidative stress, immunity, and inflammation. Int J Mol Sci. 2023;24(4):4004.
- [41]Malenica M, Prnjavorac B, et al. Effect of cigarette smoking on haematological parameters in healthy population. Med Arch. 2017;71(2):132–6.
- [42]Giustina AD, Danielski LG, et al. Vitamin B6 reduces oxidative stress in lungs and liver in experimental sepsis. An Acad Bras Cienc. 2019;91(4):e20190434.
- [43]Shan MR, Zhou SN, et al. Vitamin B6 inhibits macrophage activation to prevent lipopolysaccharide-induced acute pneumonia in mice. J Cell Mol Med. 2020;24(5):3139–48.