Antiviral potential of terpenoids against major viral infections: Recent advances, challenges, and opportunities
Abstract
Terpenoids are a diverse class of phytochemicals derived from isoprene units that have shown significant potential as antiviral agents. This review aimed to comprehensively explore the antiviral activities of terpenoids and their implications in the field of virology. Key findings from various studies have demonstrated the efficacy of terpenoids against a wide spectrum of viruses, encompassing both enveloped and non-enveloped viruses. Mechanistic insights into their antiviral action involve interference with viral entry, replication, and modulation of the host immune responses. Their multifaceted mechanisms of action make them attractive targets for combating viral infections and offer opportunities for synergy with existing antiviral drugs. Despite substantial progress in this field, several gaps must be addressed, including elucidation of specific molecular targets and signaling pathways of different terpenoids, investigations of its safety and potential side effects, and optimization of formulations and delivery methods for better bioavailability and pharmacokinetics. In conclusion, this comprehensive review provides a foundation for continued exploration and innovation in terpenoid-based antiviral research with the ultimate aim of improving global health outcomes in the face of viral threats.
INTRODUCTION
Infectious disease epidemics leading to pandemics often result from viral infections [1]. In December 2019, multiple reports of severe pneumonia emerged, and initial cases in China were attributed to a novel virus, Severe Acute Respiratory Syndrome Coronavirus 2 (SARS-CoV-2), which causes COVID-19, as identified by the World Health Organization [2].
Pandemics have a significant effect on global health and can disrupt political, social, and economic stability. Widespread infection has resulted in a large number of cases and fatalities. Measures such as travel restrictions, school and market closures, and sector-specific shutdowns indirectly influence the economic, social, and security aspects [3,4]. Addressing pandemics is a global concern, and countries are actively striving to overcome these challenges. The development of antivirals, particularly terpenoids from natural or synthetic sources, has garnered considerable attention as a means to expedite recovery and return to normal conditions [4].
Terpenoids are prevalent in microorganisms, marine organisms, plants, insects, and fungi. Various terpenoids and isomers are found in this class. Research has highlighted their diverse pharmacological effects and significant biological activities, particularly their potential as hypoglycemic, anti-inflammatory, antiviral, and antitumor agents [5]. Recent studies have increasingly emphasized the potent antiviral properties of terpenoids [6]. These compounds demonstrate the ability to hinder viral adsorption and invasion into host cells during early stages of infection, consequently impeding viral replication after cell entry [5].
This review comprehensively examines the antiviral potential of terpenoids and focuses on their mode of action against HIV, influenza viruses, hepatitis viruses, herpesviruses, coronaviruses, and other viral infections. This review also discusses the classification, biosynthesis, extraction, analysis, and improvement of terpenoid production to support its utilization in a wide range of pharmaceutical applications.
TERPENOIDS: AN OVERVIEW
The term "terpene" was first coined in 1866, deriving from the Latin word "turpentine" (Balsamum terebinthinae), which denotes a liquid extract obtained from pine trees. Terpenes, which is the largest class of natural compounds, demonstrate extensive structural diversity, that includes both linear hydrocarbons and carbocyclic skeletons [7]. Terpenoids are formed by the oxygenation, hydrogenation, or dehydrogenation of terpenes. Advancements in spectroscopic and chromatographic approaches greatly contributed to the rapid discovery of novel terpenoids [8].
Terpenoids are isoprene-derived compounds that play crucial parts in the cellular metabolism of all living organisms [9]. In plants, terpenoid diversity is abundant, with many terpenoids functioning as secondary metabolites. These specialized plant terpenoids serve as non-essential compounds and are involved in various ecological interactions between animals and plants [10]. They act as allelochemicals, attracting pollinators, deterring herbivores, or luring herbivore predators [11].
The number of identified terpenoids is extensive (Figures 1 and 2), and many are likely yet to be characterized. Terpenoids play critical roles in various biological processes in all living organisms [12]. They are involved in electron transport within the respiration chain and contribute to the biosynthesis and stability of cell walls and membranes [13]. Terpenoids are indispensable for photosynthetic organisms because they facilitate the conversion of light into chemical energy and are crucial for the function of photosynthetic reaction centers. Plant terpenoids are known to have essential functions in stress responses and defense mechanisms [13].
The broad spectrum of bioactivities of terpenoids makes them highly valuable in cosmetics, pharmaceuticals, disinfectants, colorants, agrichemicals, fragrances, and flavorings. Various terpenoids have been utilized to promote human health, such as betulinic acid (anti-HIV-1), parthenolide (tumor cell growth reduction), artemisinin (antimalarial), and taxol (anticancer) [14]. Additionally, carotenoids, including astaxanthin and lycopene, have been studied for their potential health benefits and disease-treatment properties [15]. The antiviral activities of the terpenoids are summarized in the next section.
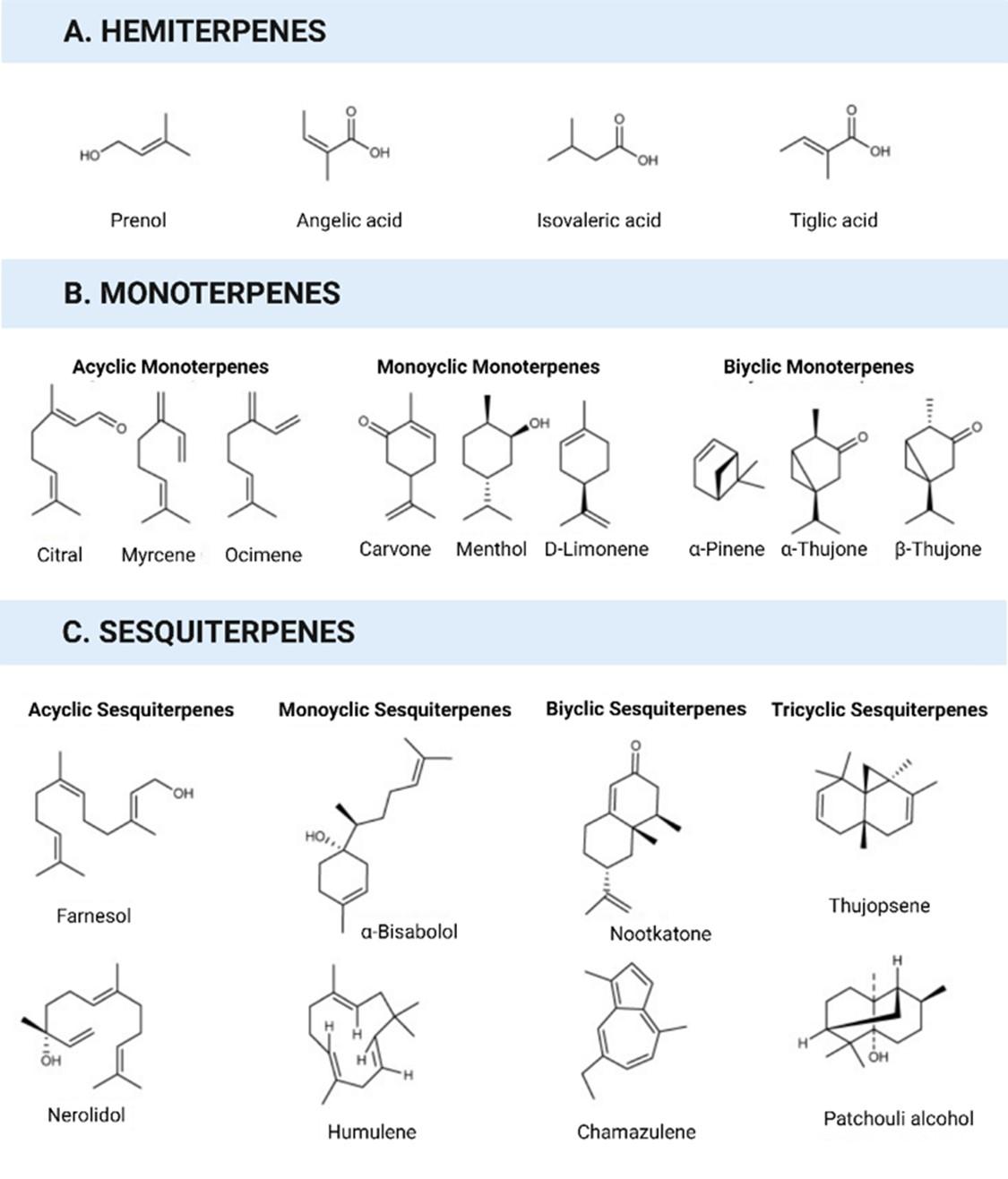
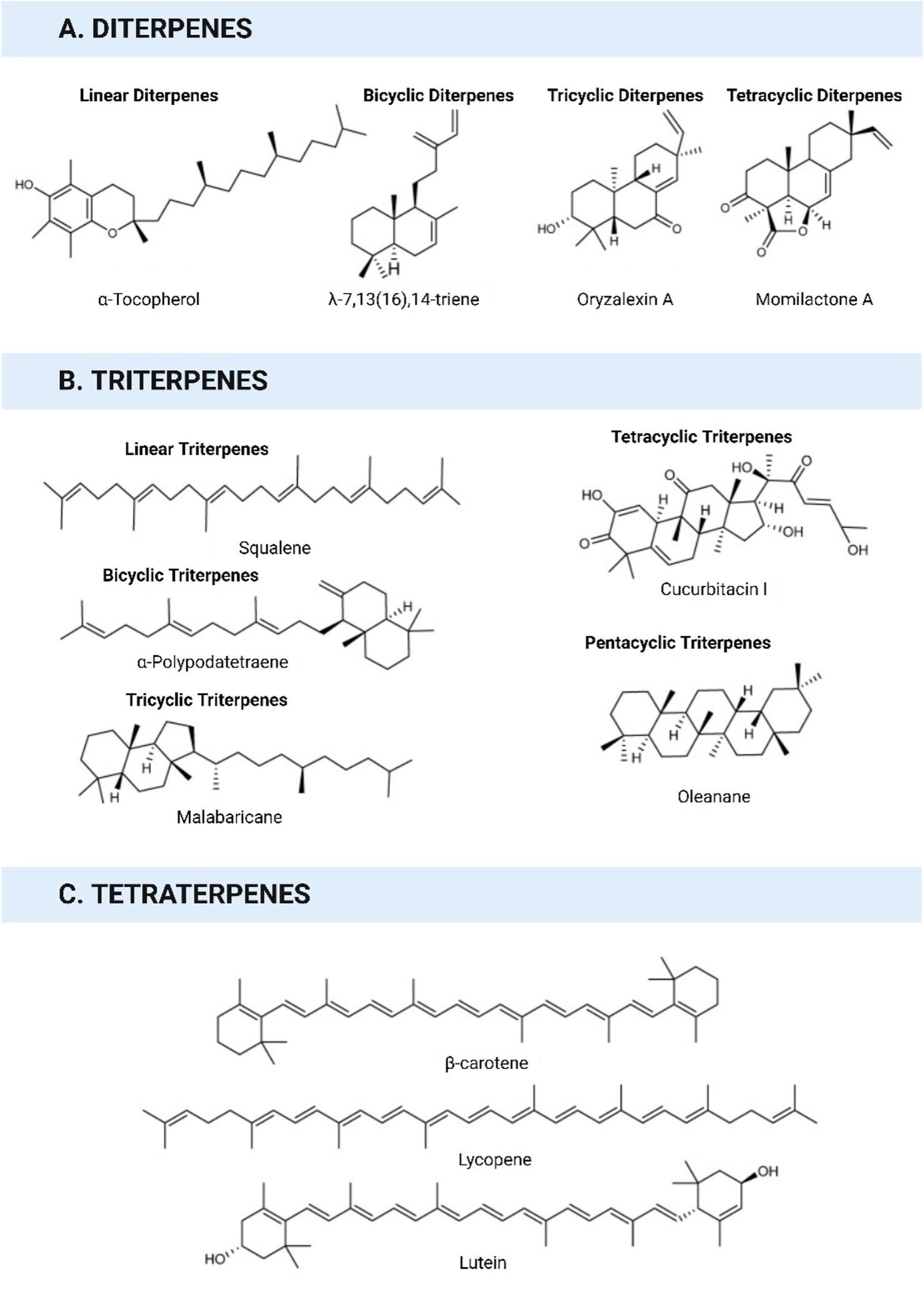
INHIBITORY MECHANISMS OF TERPENOIDS AGAINST MAJOR VIRAL DISEASES
Anti-HIV activity of terpenoids
Human Immunodeficiency Virus (HIV) primarily targets the host immune system and potentially leads to AIDS. The epidemic has affected millions of people, with millions succumbing to AIDS-related complications [16]. HIV is a retrovirus belonging to the genus Lentivirus, with a spherical shape. It contains two copies of single-stranded RNA that can be transcribed within the host [17]. There are two types: HIV-1, prevalent worldwide, and HIV-2, found mainly in West Africa and India [18]. Some terpenoid compounds, both from natural and synthetic sources, have shown potential anti-HIV activities (Figure 3).
Terpenoid compounds derived from the Euphorbiaceae species have shown anti-HIV-1 and anti-HIV-2 activities by inhibiting viral replication. Various phorbol ester derivatives, including compounds 26-40, exhibited anti-HIV-1 potential (IC50 range: 0.9 - 27.4 μM), with compound 29 being the most potent. Additionally, 12-deoxyphorbol derivatives (compounds 41-43) displayed anti-HIV-1 potential (IC50 range: 0.3 - 1.9 μM) as well as ingerol ester derivatives (202 and 203) (IC50: 17 and 27 nM, respectively). The anti-HIV activity is believed to involve the activation of protein kinase C isoenzymes (PKC). Additionally, anti-PKC beta antibodies inhibited the activation of NF-ĸB and HIV-1 induced by phorbol-12-myristate acetate (44) [19].
Terpenes obtained from Hyattella intestinalis sponge, namely spongiatriol and isospongiatriol, exhibited significant inhibition of HIV replication by acting as NF-κB inhibitors [20]. Damsin and canrenone from ethanolic extracts of Artemisia campestris subsp. glutinosa (Besser) Batt. also demonstrated anti-HIV-1 potential in vitro by blocking viral entry and interference with NF-KB and Sp1 [21] but require further studies to identify their specific mode of action.
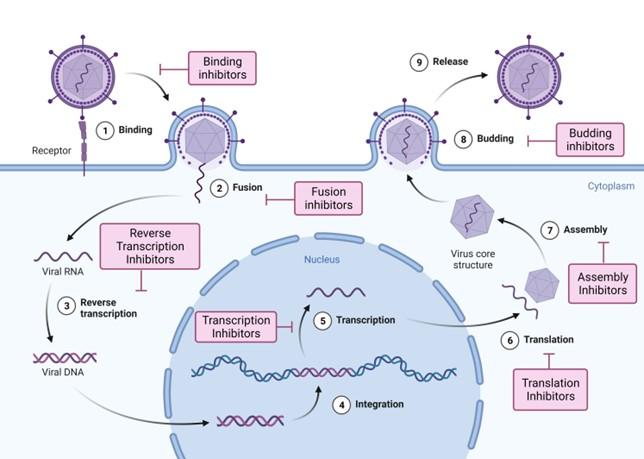
Anti-influenza virus activity of terpenoids
Influenza viruses are RNA viruses belonging to the Orthomyxoviridae family, with diverse antigenic characteristics. They are common viruses that cause severe infections in animals and humans. Influenza A and B viruses are the major culprits behind epidemics and outbreaks, significantly impacting public health and the economy [22,23]. In contrast, influenza C usually leads to sporadic mild upper respiratory symptoms [24].
Glycyrrhizin (1), obtained from licorice roots, has a broad-spectrum antiviral activity [25]. Based on 1, several derivatives were synthesized, and compounds 36–38 displayed potent anti-influenza activity (IC50 range: 4.3 - 7.2 μM) [26]. Additionally, a novel triterpenoid saponin, uralsaponin M (40), extracted from Glycyrrhiza uralensis, exhibited inhibitory action against influenza virus A/WSN/33 (H1N1) [27] but requires further validation. Three terpenoids isolated from the leaves of Euphorbia leucocephala namely, hollongdione, 3-acetoxy-lup-12,20(29)-diene, and β-amyrin acetate demonstrated strong anti-influenza activity [28].
In light of the numerous antiviral effects of PTs, a research team devised a drug screening model to evaluate a collection of PT glycoconjugates for their potential in combating influenza virus [29,30]. Through a plaque formation inhibition assay, we identified two promising compounds, the acetylated galactose–OA conjugate Y3 (43) and the acetylated galactose–UA conjugate Y5 (44), both displaying excellent anti-WSN activity (IC50: 5 μM). Compound 43 demonstrated a broad antiviral spectrum (IC50 range: 2.72 - 7.41 μM). Notably, compound 43 did not induce resistance to influenza viruses, an essential aspect for combating antiviral drug resistance.
Through the design and synthesis of multivalent PT‐α‐(β‐, γ‐) CD conjugates (45–48), potency against the influenza virus was improved [31]. Among these conjugates, multivalent OA‐β‐CD conjugate 46 exhibited the strongest antiviral potential against influenza A virus (IC50:1.6 μM). The multivalent conjugates have the ability to bind with the influenza viral HA, thereby preventing the attachment to host cells.
Anti-SARS-CoV2 activity of terpenoids
SARS-CoV-2, which has caused the recent outbreak and COVID-19 epidemic, is highly infectious and transmissible. The pathogenesis of SARS-CoV-2 infection starts upon entry into the epithelial cells of the oral and nasal mucosa upon viral attachment to host cell receptors, through the viral spike protein. The spike protein, a protrusion on the virion’s surface, acts as the primary cell recognition system and entry [32]. Upon entry, it hijacks host cellular machinery for genome replication, transcription, assembly, and proliferation. Within these stages, key proteins and enzymes play vital roles in the pathogenicity, virulence, and infectivity of SARS-CoV-2 [33]. Among these are the structural spike protein and non-structural enzymes, such as RNA-dependent RNA polymerase (RdRp), papain-like protease (PLpro), and 3-chymotrypsin-like protease (3CLpro or Mpro) [34].
Santos et al. (2022) investigated proprietary formulations, which included a combination of cannabidiol (52) and terpenes extracted from Origanum vulgare, Thymus mastichina, and Cannabis sativa L., to reduce SARS-CoV-2 infectivity. The virucidal and antiviral potentials of terpene-based formulations were assayed in vitro in several cell lines. Formulation F2TC was found to reduce infectivity by 99% in A549 cells, while the FITC formulation reduced infectivity by 43% in the HEK293T cell line [35].
Artemisinins, a group of antimalarial sesquiterpene lactone drugs, have recently been explored as potential inhibitor for SARS-CoV-2. An in vitro assay was conducted to evaluate the antiviral activity. The results of their study revealed that arteannuin B (53) exhibited the strongest anti-SARS-CoV-2 potential followed by artesunate (54) and dihydroartemisinin (55) [36]. Zhou et al. (2021) performed additional studies on the potential of several artemisinins against SARS-CoV-2, particularly artesunate (54), artemisinin (56), artemether (57), and Artemisia annua extracts. In the concentration-response antiviral treatment assay, which is a SARS-CoV-2 spike glycoprotein immunostaining in vitro assay, artesunate (54) showed to be the most potent against different cell types with an EC50 range of 7-12 μg/mL. Compound 54 was also shown to inhibit SARS-CoV-2 at after viral entry, based on time-of-addition experiments [37]. Hu et al. provided further validation of the inhibitory activity of artemisinins against SARS-CoV-2. The team screened three sesquiterpene lactone derivatives, arteannuin B (53), artesunate (54), and artemether (57), along with other monomeric chalcones, that displayed excellent anti-SARS-CoV-2 activities [38].
Diterpenes tanshinone IIA (58) and carnosic acid (59) also displayed inhibitory activity against SARS-CoV-2 when tested in vitro via plaque reduction assay. In the same study, they subjected the compounds to molecular docking analysis and molecular dynamics simulations and found that tanshinone IIA (58) showed stable complexes with SARS-CoV-2 Mpro and spike proteins [39].
Another possible strategy to inhibit SARS-CoV-2 is the downregulation of the expression or inhibition of host receptor proteins such as ACE2 [40]. Kumar et al. (2020) investigated the ACE2 inhibitory potential of several essential oils and their major compounds. These results suggested that the monoterpenoids citronellol (60) and limonene (61), the major components of lemon essential oils and geranium, respectively, significantly suppressed ACE2 synthesis in epithelial cells [41].
Anti-hepatitis virus activity of terpenoids
Diverse hepatitis viruses, the causative agent of viral hepatitis, are classified into types A, B, C, D, and E. Hepatitis A and E viruses lead to acute infections that are transmitted through the fecal-oral pathway. Unlike hepatitis A, hepatitis B, C, and D viruses cause chronic infections and are transmitted through blood and bodily fluids [42]. Terpenoids have gained attention for treating viral hepatitis [43], which is discussed in this section.
Chronic hepatitis B (CHB) infection is a persistent, long-term illness that occurs as a consequence of HBV infection, ultimately leading to liver problems such as hepatitis, cirrhosis, and cancer [44]. The current treatment protocol incorporates the use of both IFN and nucleoside drugs, including adefovir dipivoxil, clevudine, and lamivudine. Despite the potential benefits, the limited success rate of treatment, the resistance to nucleoside-based drugs, and the severe side effects of interferon have hindered their widespread use in clinical settings [42].
Three new friedelolactones isolated from Viola diffusa Ging, including violalide, violaic A, and violaic B, were found to inhibit HBsAg and HBeAg secretion [45]. The cleavage-A-pentacyclic triterpene derivatives exhibited significantly increased inhibitory effects on HBeAg secretion compared to the parent compound OA (8) [46]. Similar to lamivudine, GL-3, a derivative of GL (1), showed strong inhibition HBV DNA replication. Astragaloside IV (AS-IV, 49) demonstrated effective anti-HBV effects by viral DNA replication and inhibiting cell proliferation [47]. Additionally, G-Rg3 (31) demonstrated suppression of viral genome replication and protein synthesis, whereas G-Rg1 (32) improved the immune response to an anti-HBsAg vaccine, exerting an anti-HBV effect [48].
Chronic hepatitis C (CHC) affects approximately 185 million people, leading to liver cirrhosis and liver cancer, which are common complications of HCV infection. NS3/4A serine protease is involved in HCV replication by cleaving polyprotein precursors and facilitating the maturation of nonstructural proteins. At present, more than 95% of CHC patients are being treated with drugs that target NS3/4A proteases, resulting in a sustained decrease in viral levels. Despite this, several liver cirrhosis patients develop hepatocellular carcinoma and drug resistance, and side effects continue to be significant challenges [49].
Oleanolic acid (OA), an active ingredient of Dipsacus asperoides, has demonstrated significant anti-HCV activity [50]. Its derivative, echinocystic acid (EA), also exhibits activity against HCV by suppressing the binding interactions of HCV E2 protein to CD81 thereby preventing HCV from being recognized by the host [51].
Terpenoids isolated from Artocarpus heterophyllus leaves by inhibiting the post-viral entry stages such as HCV NS3 protein expression and HCV RNA replication with marginal effects on the viral entry stage. Synergistic effect was also observed when A. heterohyllus extract was combined with commercial anti-HCV drugs; Ribavirin, Simeprevir, Cyclosporin A [52].
NS5b is essential for HCV RNA synthesis and replication, facilitating the replication of HCV RNA genome [53]. Both OA and ursolic acid (UA) suppress NS5B and RNA polymerase activities [53]. Additionally, G-Rg3 (31) inhibits HCV-infected cell proliferation by regulating p21 expression, restoring mitochondrial division, and suppressing HCV replication [54].
Anti-rotavirus activity of terpenoids
Rotavirus (RV) stands as a major contributor to severe enteritis in young children, as it infects and damages the cells of the intestinal mucosa, triggering the release of harmful toxins that can cause malnutrition and, in extreme cases, death. At present, no antiviral drug is available for RV. However, 2α-Hydroxyursolic acid showed potent anti-RV activity by stimulating IFN-γ secretion, thereby enhancing immune function [55]. Additionally, UA inhibits RV replication and interferes with viral particle maturation [56]. Furthermore, 20(S)-GRg3 and G-Rb2 act as immunomodulators and display effective anti-RV effects [57]. Strong in vitro anti-rotaviral activity from terpenes isolated from Achyrocline bogotensis (Kunth) DC was also reported with low intestinal toxicity [58].
Anti-herpesvirus activity of terpenoids
Herpes simplex virus (HSV) activity of terpenoids
The herpes simplex virus (HSV), which is a member of the Herpesviridae family, presents significant public health challenges. HSV-1 affects a substantial portion of the global population under the age of 50 [59]. FDA-approved drugs, such as acyclovir and its derivatives, are utilized for HSV treatment [60]. Nevertheless, their use is restricted owing to harmful side effects and antiviral drug resistance [61]. Therefore, there is a demand for innovative HSV inhibitors.
The medicinal plant Rhus javanica has demonstrated anti-HSV activity [62]. Two major compounds from this plant, betulonic acid (66) and moronic acid (74), were isolated, and their anti-HSV potentials were assessed in vitro and in vivo [63]. Compound 66 showed an effective concentration resulting in 50% plaque reduction of HSV-1 at 5.7 μM, whereas compound 74 exhibited this effect at 8.6 μM [62].
Ikeda et al. conducted a study of 15 oleanane-type triterpenoids to evaluate their anti-HSV-1 potential [64]. Results revealed that sapogenol of glycyrrhizin (1) and glycyrrhetic acid (2) exhibited higher anti-HSV-1 potency compared to glycyrrhizin (1). Soyasapogenol A had weaker antiviral activity against HSV-1 than soyasapogenol B, indicating that hydroxylation at C-21 may decrease anti-HSV activity [64].
Gong et al. conducted a drug combination study to investigate the synergistic antiviral activity of acyclovir and betulin against HSV-1 [65]. The researchers found that betulin and acyclovir exhibited strong synergistic anti-HSV-1 effects at concentrations higher than 4.4 μM and 0.9 μM, respectively. Additionally, synergistic anti-HSV-2 effects were observed at concentrations of 2.0 μM Acyclovir and 19.0 μM betulin. This suggests that the mechanism of action of betulin differs from that of Acyclovir.
Cytomegalovirus (CMV) activity of terpenoids
Cytomegalovirus (CMV) belongs to the Herpesviridae family and is the largest virus in the Betaherpesvirinae subfamily. Current treatment options for CMV include DNA polymerase inhibitors including acyclovir, ganciclovir, foscarnet, and cidofovir. However, no single drug provides sufficient potency and safety, and the emergence of ganciclovir resistance mutations has been reported. These resistance mutations may also lead to cross-resistance to other antiviral drugs [66].
Current therapeutic targets for antiviral drugs against CMV include viral terminase complex inhibitor, viral kinase UL97 inhibitor, and viral DNA polymerase UL54, for which commercial drugs are available [67]. Additionally, therapies targeting CMV latency have been suggested to address the reactivation of latently infected cells mediated by myeloid differentiation and pro-inflammatory signals [66]. During latency, inhibitors of the viral replicase are inefficient due to the lack of viral replication, and the CMV protein US28 is expressed, offering a potential therapeutic target [68]. Despite limited reports, identifying promising anti-CMV terpenoids is essential to effectively target both replication and latency stages. Further studies are warranted in this regard.
Anti-dengue virus activity of terpenoids
Dengue virus (DENV) from Flaviviridae family is the causative agent of a range of diseases from dengue-to-dengue shock syndrome [69]. It is prevalent in tropical regions and consists of five types: DENV 1-5 [70] with approximately 390 million cases annually [71]. The number of dengue fever cases and related deaths has been on the rise, with reported cases increasing from 505,430 (year 2000) to 4.2 million (year 2019), and deaths increasing from 960 (year 2000) to 4032 (year 2015) [72] further necessitating more research efforts to find the next potent anti-DENV drug.
Andrographolide demonstrated inhibitory activity against DENV-2 [73] associated with GRP78 and unfolded protein response [74]. Trigocherrin A (15) and trigocherriolides A-B (18–19) from Trigonostemon cherrieri exhibit inhibitory effects against DENV, with IC50 values ranging from 3.1 16.0 μM [75]. Additionally, (+)-ferruginol (63) and 18-oxoferruginol (62) show anti-DENV activity, with IC50 of 1.4 and 5.0 μM, respectively [76].
Anti-zika virus activity of terpenoids
Zika virus (ZIKV) is a member of the genus Flavivirus under the family Flaviviridae. It was initially identified in non-human primates in 1947, and later in mosquitoes and humans in 1948 and 1952, respectively [77]. There are similarities in the clinical presentation of ZIKV, chikungunya, and dengue, and these viral diseases are transmitted by mosquitoes. In 2007, an outbreak occurred in the South Pacific, which was followed by another epidemic in 2013–2014. During these events, neurological complications related to the infection were identified for the first time [78]. Currently, vaccine is still under development, and the CDC recommends avoiding Aedes mosquito bites as the best preventive measure against ZIKV [77].
Recently, the emergence of ZIKV outbreaks has spurred research into drug development, leading to the synthesis of various synthetic compounds with anti-ZIKV potential. Abreu et al. isolated diterpenoids and loranthones A-D from Stillingia loranthacea. Among them, loranthone B demonstrated significant antiviral activity, reducing ZIKV replication by 1.7 log10 TCID50/mL against a ZIKV strain from Brazil [79].
Anti-chikungunya virus activity of terpenoids
Chikungunya virus (CHIKV) is a member of the family Flaviviridae and is also transmitted by the Aedes aegypti mosquito. The duration of CHIKV's incubation period is relatively brief, ranging from 2 to 4 days. This virus presents symptoms like high fever, joint pain, and a facial rash. CHIKV infection has been reported in various regions of Asia, Europe, America, and Africa [80].
Several terpenes have demonstrated antiviral activities against CHIKV. Trigonostemon cherrieri-derived daphnane diterpenes, including trigocherriolides A-C, trigocherrins A-B, and trigocherrin F, exhibit stronger inhibition of CHIKV replication than chloroquine [75]. Moreover, tonantzitlolone C, tonantzitlolone F, and tonantzitlolone B also demonstrated significant CHIKV inhibition [81].
The marine cyanobacterium Trichodesmium erythraeum produces several terpenes with anti-CHIKV activities, including anhydrodebromoaplysiatoxin, 3-methoxydebromoaplysiatoxin, and debromoaplysiatoxin. Debromoaplysiatoxin exhibited superior inhibition of CHIKV infection compared with anhydrodebromoaplysiatoxin and 3-methoxydebromoaplysiatoxin. These terpenes inhibit viral DNA polymerase, a crucial enzyme in the viral replication after entering the host cell [82]. Additionally, Canistrocarpus cervicornis-derived dolastane demonstrated potent inhibitory activity against CHIKV (EC50: 1.28 μM) [83].
Anti-norovirus activity of terpenoids
Norovirus (NoV) is a significant contributor to non-bacterial acute gastroenteritis, commonly in infants and young children [84]. NoV represents a significant global health risk, responsible for over half of viral gastroenteritis patients and more than 85% of non-bacterial gastroenteritis patients worldwide [85]. The discovery of NoV inhibitors has made substantial progress, and the exploration of natural products for compounds with antiviral potential against NoV is a commendable approach. Recently, astragaloside was identified as an anti-NoV agent that effectively reduces the expression of TNF-α, IL-1β, and IL-6, enhances patients' anti-inflammatory responses, and promotes the elimination of viruses by intestinal immune cells [86]. Propolis-derived terpenoids also showed antiviral potential against human norovirus GII.4 by denaturing the viral capsid protein which may have prevented viral adsorption and internalization to host cells [87].
Anti-coxsackie virus V3 activity of terpenoids
Coxsackievirus B3 (CVB3) is a serotype of the coxsackievirus within the family Picornaviridae, specifically the Enterovirus genus. CVB3 is a type of virus that is transmitted through the gastrointestinal route and is identified by non-enveloped, positive-sense, and single-stranded RNA. Notably, terpenoids have demonstrated beneficial effects in viral myocarditis [88].
Various Lyonia ovalifolia-derived ursane, lanostane, and cycloartane triterpenes were purified and assessed their effects against CV B3. Among these compounds, lyonifolosides A-K and their aglycones were investigated, with lyonifolic acid A, lyonifoloside A, lyonifoloside B, and lyofoligenic acid, demonstrating potent activity against CVB3 [89].
The IC50 values for these cycloartane compounds indicate that those with an olefinic bond at the 5(10) positions are more active than those with a bond at the 1(10) positions. Additionally, aglycones demonstrated greater potency than their glycoside counterparts, highlighting the possible importance of C-3 hydroxyl group [89].
Lv et al. (2016) reported lanostane triterpenoids (28–35) from Lyonia ovalifolia's leaves and twigs. Among these, lyonifolic acid C (29) and lyonifolic acid L (28) showed potent anti-CVB3 activity. The presence of the C-21 position was found to be crucial for its antiviral mechanism since glycosylation resulted in the weakening of the antiviral effect. Additionally, the aglycone showed higher potency than its glycoside counterpart, while acetylation of the attached sugar enhanced its antiviral effect [89].
Anti-enterovirus 71 activity of terpenoids
Enterovirus 71 (EV71) is a member of Picornaviridae family containing a non-enveloped positive sense ssRNA virus. EV71 is responsible for hand, foot, and mouth disease (HFMD) with potentially severe outcomes such as acute flaccid paralysis (AFP) and epidemic encephalitis, leading to cardiopulmonary problems and fatalities in children. Unfortunately, no effective treatment for EV71 infections is currently available [90].
Hedera helix-derived hederasaponin B exhibits potent inhibitory activity against EV71 [91]. Hederasaponin B effectively reduced cytopathic effects (CPE) in Vero cells without causing cytotoxicity. Moreover, it demonstrated inhibitory effects on VP2 expression which directly affects capsid protein synthesis. Notably, hederasaponin B showed superior anti-EV71 activity compared to ribavirin, making it a top candidate due to its broad-spectrum anti-EV71 properties [91].
The anti-EV71 activity of six newly synthesized PTs were also investigated [92]. The inhibitory effects on VP1 protein expression were assessed through RT-PCR and western blot analysis. Compounds 85, 86, and 87 exhibited stronger anti-EV71 activities than their parent compounds and did not cause any significant cytotoxicity.
Anti-respiratory syncytial virus activity of terpenoids
Respiratory syncytial virus (RSV) is a member of the Pneumoviridae family and is responsible for respiratory illnesses in infants and elderly individuals. Aerosolized ribavirin, palivizumab, and motavizumab are approved treatments, while anti-RSV drug development is ongoing [93]. Natural products are being explored for their anti-RSV activities, including terpenes, although limited studies have been conducted in this area [94]. As such, Lilium speciosum var. gloriosoides-derived (20R)-20,25-epoxy-3-methyldammaran-2-en-6α,12β-diol demonstrated strong anti-respiratory syncytial virus (RSV) activity whereas other compounds showed moderate to weak inhibition [95].
Anti-epstein-barr virus activity of terpenoids
Epstein-Barr virus (EBV) causes infectious mononucleosis in humans and is also linked to nasopharyngeal carcinoma and Burkitt's lymphoma in endemic regions. The prevalence of EBV-related illnesses is growing, emphasizing the need for novel antiviral agents for its prevention and treatment [96,97].
In a study by Lin et al., glycyrrhizin demonstrated dose-dependent activity against EBV replication (IC50 = 0.04 mM, CC50 = 4.8 mM, SI = 120) [98]. The inhibitory effect of glycyrrhizin was attributed to post-viral entry, indicating that its mechanism is distinct from inhibitors of EBV’s DNA polymerase (i.e., nucleoside analogs). These findings suggest that glycyrrhizin and its derivatives are potential novel anti-EBV agents that target the early steps in the EBV replicative cycle [98].
Chang and colleagues initially identified moronic acid (76) from Rhus chinensis and Brazilian propolis as an effective compound with anti-EBV activity [96]. It targets Rta, a critical transcription factor of EBV, inhibits its function, and reduces the expression of Zta and EA-D proteins. This novel mechanism makes compound 76 a promising candidate for anti-EBV drug development (EC50 = 3.15 μM, CC50 = 46.67 μM, SI = 14.8) [96].
Anti-porcine reproductive and respiratory syndrome virus activity of terpenoids
Porcine reproductive and respiratory syndrome virus (PRRSV) poses a serious global threat to pig populations and available vaccines offer limited immunity. GL (1) has shown promise as an inhibitor of PRRSV by hindering cellular penetration and reducing viral proliferation and protein expression [99,100]. Moreover, Gly CDs derived from GL (1) exhibit antiviral effects against PRRSV by stimulating host innate immunity, reducing intracellular reactive oxygen species accumulation, and regulating the synthesis of NOS3 and DDX53 to impede viral replication [101]. These findings suggest that GL and its derivatives are potential candidates for combating PRRSV infection.
G-Rg1 (32) demonstrated potent inhibition of PRRSV replication in both PAMs and MARC-145 cells [102]. Its inhibitory effects encompass various PRRSV life cycle stages. Notably, G-Rg1 (32) exhibited broad anti-PRRSV activity, including XH-gD, NADC-30, JXA1, and classic strain VR2332. In addition to its antiviral actions, G-Rg1 (32) downregulated pro-inflammatory cytokines and mitigated NF-κB pathway activation induced by PRRSV. Furthermore, a UA derivative, specifically compound 15, displayed direct inactivation of PRRSV viral particles, leading to effective suppression of PRRSV genome replication and exerting antiviral effects [103]. These findings highlight the potential of G-Rg1 (32) and the UA-derived compound 15 as promising candidates for combating PRRSV infections.
Anti-porcine epidemic diarrhea virus activity of terpenoids
Porcine epidemic diarrhea virus (PEDV) causes significant mortality in the global swine population. It is classified under the Pedacovirus subgenus within the Alphacoronavirus genus and Coronaviridae family. PEDV is characterized by its positive-sense, enveloped, single-stranded 28 kb RNA genome [104]. Although it was first identified in China in 1980, its impact became more pronounced in 2010, during a large outbreak that caused substantial economic concerns [105,106]. Subsequently, it emerged in the USA in late 2013 and has become a global presence [107].
In the quest for drug development against PEDV, researchers are exploring various targets, such as kinase inhibitors, 3CL protease, and main protease [108]. Notably, natural products such as quercetin and homoharringtonine, along with plant extracts, have demonstrated inhibitory activity against PEDV, highlighting the antiviral potential of oleanane triterpenes [109].
STRATEGIES TO IMPROVE TERPENOID PRODUCTION
The cellular regulation of terpenoid production is crucial, and the maximum productivity is determined by rate-limiting steps in the MEP pathway. Recent studies have attempted to overcome these limitations in cyanobacterial model organisms. DXS, the enzyme responsible for the first step of the MEP pathway, has been identified as a bottleneck [110]. Overexpression of DXS in Synechocystis led to increased carotenoid levels, suggesting that optimizing gene expression and restricting synthesis of storage compounds could enhance terpenoid yield in cyanobacteria [111]. However, another study in Synechococcus sp. PCC 7002 showed no increase in terpenoid production when glycogen synthesis was inactivated, but rather observed the secretion of central metabolites unrelated to terpenoid biosynthesis [112].
In another recent study, overexpression of native enzymes DXS, IDI, and GDPS in Synechocystis led to a 1.4-fold increase in limonene production, similar to the effect on carotenoid formation observed in a previous study with overexpression of dxs alone [113]. Similarly, in a study on a limonene-producing strain of Anabaena sp. PCC 7120, heterologous overexpression of DXS from E. coli, IDI from Haematococcus pluvialis, and GDPS from Mycoplasma tuberculosis resulted in a 2.3-fold increase in limonene production under low light and a remarkable 6.8-fold increase under high light conditions. Additionally, it was observed that limonene-producing strains exhibited higher oxygen evolution under high light, indicating limonene production acted as an extra carbon sink in the cells [113]. These findings suggest the potential advantage of using heterologous enzymes to enhance carbon flux through the MEP pathway, potentially avoiding interference from native enzyme regulation on their activity.
In a more advanced approach, as described earlier, Bentley et al. successfully performed heterologous expression of the entire MEV pathway, controlled by the psbA2 promoter, in an isoprene-producing strain of Synechocystis, resulting in a remarkable 2.5-fold increase in isoprene yield. The enhanced product formation is attributed to the availability of additional substrate for terpenoid synthesis, as the MEV pathway utilizes acetyl-CoA instead of GAP and pyruvate [114]. However, the observed increase in yield may still be constrained by potential bottlenecks in the introduced pathway.
CONCLUSIONS
Terpenoids exhibited potent antiviral activities against a wide spectrum of human and animal viruses. Their diverse structural and chemical properties offer promising opportunities for developing novel antiviral agents. Various classes of terpenoids, including monoterpenes, sesquiterpenes, diterpenes, and triterpenes, exhibit potent inhibitory effects on viral replication and entry processes.
Despite these promising results, there are still some research gaps that need to be addressed. First, more in-depth investigations are necessary to elucidate the mechanisms of action of different terpenoids against specific viruses. Explorations into the safety and potential side effects of these compounds are crucial for their clinical application. Furthermore, optimization of terpenoid formulations and delivery methods for better bioavailability and pharmacokinetics requires further exploration.
Researchers should continue to explore novel natural sources to discover new terpenoid compounds with potent antiviral properties. Moreover, the combination of terpenoids with other antiviral agents or conventional drugs may lead to synergistic effects and improve therapeutic outcomes. Advancements in biotechnology and metabolic engineering can also contribute to enhancing terpenoid production through synthetic biological approaches. By bridging gaps in knowledge, conducting rigorous studies, and leveraging innovative approaches, terpenoids could revolutionize antiviral therapy in the future.
ACKNOWLEDGMENTS
The authors would like to thank the DOST S&T Fellows Program; the Philippine Council for Agriculture, Aquatic and Natural Resources Research and Development (DOST-PCAARRD) for funding this research project; and the Industrial Technology Development Institute (DOST-ITDI) for hosting this research project. This project was funded by the Department of Science and Technology - Philippine Council for Agriculture, Aquatic and Natural Resources Research and Development (DOST-PCAARRD).
AUTHOR CONTRIBUTIONS
FLO: Conception and design of the study, wrote the first draft of the manuscript, critically revised the manuscript, funding acquisition. MTJQ: wrote the first draft of the manuscript, and revised the manuscript. All authors approved the final version of the manuscript.
CONFLICTS OF INTEREST
There is no conflict of interest among the authors.
References
- [1]Huremović D. Brief history of pandemics (pandemics throughout history). In: Huremović D, editor. Psychiatry pandemics ment. health response Infect. Outbreak. Cham: Springer International Publishing; 2019. p. 7–35.
- [2]Lai C-C, Shih T-P, et al. Severe acute respiratory syndrome coronavirus 2 (SARS-CoV-2) and coronavirus disease-2019 (COVID-19): The epidemic and the challenges. Int J Antimicrob Agents. 2020;55:105924.
- [3]Mahedi MRA, Rawat A, et al. Understanding the global transmission and demographic distribution of nipah virus (niv). Res J Pharm Technol. 2023;16:3588–3594.
- [4]Qiu W, Rutherford S, Mao A, Chu C. The pandemic and its impacts. Health Cult Soc. 2017;9:1–11.
- [5]Xu C, Wang B, et al. Techniques for the analysis of pentacyclic triterpenoids in medicinal plants. J Sep Sci. 2018;41:6–19.
- [6]Li H, Sun J, et al. Triterpenoid-mediated inhibition of virus–host interaction: Is now the time for discovering viral entry/release inhibitors from nature? J Med Chem. 2020;63:15371–15388.
- [7]Abdallah II, Quax WJ. A glimpse into the biosynthesis of terpenoids. KnE Life Sci. 2017:81–98.
- [8]Ninkuu V, Zhang L, et al. Biochemistry of terpenes and recent advances in plant protection. Int J Mol Sci. 2021;22:5710.
- [9]Bouvier F, Rahier A, et al. Biogenesis, molecular regulation and function of plant isoprenoids. Prog Lipid Res. 2005;44:357–429.
- [10]Clavijo McCormick A, et al. The specificity of herbivore-induced plant volatiles in attracting herbivore enemies. Trends Plant Sci. 2012;17:303–310.
- [11]Masyita A, Mustika Sari R, et al. Terpenes and terpenoids as main bioactive compounds of essential oils, their roles in human health and potential application as natural food preservatives. Food Chem X. 2022;13:100217.
- [12]Fan M, Yuan S, et al. Application of terpenoid compounds in food and pharmaceutical products. Fermentation. 2023;9:119.
- [13]Anjali, Kumar S, et al. Role of plant secondary metabolites in defence and transcriptional regulation in response to biotic stress. Plant Stress. 2023;8:100154.
- [14]Mushtaq M, Anwar F. A Centum of Valuable Plant Bioactives. Academic Press; 2021.
- [15]Ahmad A, Riaz S, et al. Role of carotenoids in cardiovascular disease. Carotenoids - New Perspect. Appl. IntechOpen; 2022.
- [16]Ornos EDB, Tantengco OAG, et al. Global online interest in HIV/AIDS care services in the time of COVID-19: A Google trends analysis. AIDS Behav. 2023;27:1998–2004.
- [17]Lefkowitz EJ, Dempsey DM, et al. Virus taxonomy: the database of the International Committee on Taxonomy of Viruses (ICTV). Nucleic Acids Res. 2018;46:D708–D717.
- [18]Luzuriaga K. Early Combination antiretroviral therapy limits hiv-1 persistence in children. Annu Rev Med. 2016;67:201–213.
- [19]Nothias-Scaglia L-F, Pannecouque C, et al. antiviral activity of diterpene esters on chikungunya virus and HIV replication. J Nat Prod. 2015;78:1277–1283.
- [20]Ahmadi P, Haruyama T, et al. Spongian diterpenes from the sponge Hyattella aff. intestinalis. Chem Pharm Bull (Tokyo). 2017;65:874–877.
- [21]Ticona LA, Bermejo P, Guerra JA, et al. Ethanolic extract of Artemisia campestris subsp. glutinosa (Besser) Batt. inhibits HIV–1 replication in vitro through the activity of terpenes and flavonoids on viral entry and NF–κB pathway. J Ethnopharmacol. 2020;263:113163.
- [22]Khazeni N, Hutton DW, et al. Health and economic benefits of early vaccination and nonpharmaceutical interventions for a human influenza A (H7N9) pandemic: a modeling study. Ann Intern Med. 2014;160:684–694.
- [23]Orosco FL. Breaking the chains: Advancements in antiviral strategies to combat Nipah virus infections. Int J One Health. 2023:122–133.
- [24]Poon LLM, Song T, et al. Quantifying influenza virus diversity and transmission in humans. Nat Genet. 2016;48:195–200.
- [25]Hoever G, Baltina L, et al. Antiviral activity of glycyrrhizic acid derivatives against SARS-coronavirus. J Med Chem. 2005;48:1256–1259.
- [26]Baltina LA, Zarubaev VV, et al. Glycyrrhizic acid derivatives as influenza A/H1N1 virus inhibitors. Bioorg Med Chem Lett. 2015;25:1742–1746.
- [27]Song W, Si L, et al. Uralsaponins M-Y, antiviral triterpenoid saponins from the roots of Glycyrrhiza uralensis. J Nat Prod. 2014;77:1632–1643.
- [28]Chen H-T, Chuang C-W, et al. Terpenoids with anti-influenza activity from the leaves of Euphorbia leucocephala. Nat Prod Res. 2023;37:936–943.
- [29]Xiao S, Wang Q, et al. Synthesis and biological evaluation of novel pentacyclic triterpene α-cyclodextrin conjugates as HCV entry inhibitors. Eur J Med Chem. 2016;124:1–9.
- [30]Orosco F. Advancing the frontiers: Revolutionary control and prevention paradigms against Nipah virus. Open Vet J. 2023;13:1056–1056.
- [31]Tian Z, Si L, et al. Inhibition of influenza virus infection by multivalent pentacyclic triterpene-functionalized per-O-methylated cyclodextrin conjugates. Eur J Med Chem. 2017;134:133–139.
- [32]Quimque MTJ, Notarte KIR, et al. Computationally repurposed natural products targeting sars-cov-2 attachment and entry mechanisms. In: Adibi S, Griffin P, Sanicas M, Rashidi M, Lanfranchi F, editors. Front. COVID-19 Sci. Clin. Asp. Nov. Coronavirus 2019. Cham: Springer International Publishing; 2022. p. 505–537.
- [33]Jackson CB, Farzan M, et al. Mechanisms of SARS-CoV-2 entry into cells. Nat Rev Mol Cell Biol. 2022;23:3–20.
- [34]Gil C, Ginex T, et al. COVID-19: Drug targets and potential treatments. J Med Chem. 2020;63:12359–12386.
- [35]Santos S, Barata P, et al. Cannabidiol and terpene formulation reducing SARS-CoV-2 infectivity tackling a therapeutic strategy. Front Immunol. 2022;13.
- [36]Cao R, Hu H, et al. Anti-SARS-CoV-2 potential of artemisinins in vitro. ACS Infect Dis. 2020;6:2524–2531.
- [37]Zhou Y, Gilmore K, et al. In vitro efficacy of artemisinin-based treatments against SARS-CoV-2. Sci Rep. 2021;11:14571.
- [38]Hu Y, Liu M, et al. Artemether, artesunate, arteannuin b, echinatin, licochalcone b and andrographolide effectively inhibit SARS-CoV-2 and related viruses in vitro. Front Cell Infect Microbiol. 2021;11.
- [39]Elebeedy D, Elkhatib WF, et al. Anti-SARS-CoV-2 activities of tanshinone IIA, carnosic acid, rosmarinic acid, salvianolic acid, baicalein, and glycyrrhetinic acid between computational and in vitro insights. RSC Adv. 2021;11:29267–29286.
- [40]Ni W, Yang X, et al. Role of angiotensin-converting enzyme 2 (ACE2) in COVID-19. Crit Care Lond Engl. 2020;24:422.
- [41]Senthil Kumar KJ, Gokila Vani M, et al. Geranium and lemon essential oils and their active compounds downregulate angiotensin-converting enzyme 2 (ACE2), a SARS-CoV-2 spike receptor-binding domain, in epithelial cells. Plants Basel Switz. 2020;9:770.
- [42]Roy A, Roy M, et al. Role of bioactive compounds in the treatment of hepatitis: A review. Front Pharmacol. 2022;13.
- [43]Crance J-M, Lévêque F, et al. Studies on mechanism of action of glycyrrhizin against hepatitis a virus replication in vitro. Antiviral Res. 1994;23:63–76.
- [44]Lee MH, Lee B-H, et al. Reduction of hepatitis A Vvrus on FRhK-4 Cells treated with korean red ginseng extract and ginsenosides. J Food Sci. 2013;78:M1412–M1415.
- [45]Dai J-J, Tao H-M, Min Q-X, Zhu Q-H. Anti-hepatitis B virus activities of friedelolactones from Viola diffusa Ging. Phytomedicine. 2015;22:724–729.
- [46]Liu Y, Yang L, et al. Recent advances in antiviral activities of triterpenoids. Pharmaceuticals. 2022;15:1169.
- [47]Li L, Hou X, et al. Research review on the pharmacological effects of astragaloside IV. Fundam Clin Pharmacol. 2017;31:17–36.
- [48]Yuan D, Yuan Q, et al. Vaccine adjuvant ginsenoside Rg1 enhances immune responses against hepatitis B surface antigen in mice. Can J Physiol Pharmacol. 2016;94:676–681.
- [49]Ogawa E, Nomura H, et al. Development of hepatocellular carcinoma in patients aged 75–84 years with chronic hepatitis C treated with direct-acting antivirals. J Infect Dis. 2022;226:431–440.
- [50]Yu F, Wang Q, et al. Development of oleanane-type triterpenes as a new class of HCV entry inhibitors. J Med Chem. 2013;56:4300–4319.
- [51]Vo NNQ, Fukushima EO, et al. Structure and hemolytic activity relationships of triterpenoid saponins and sapogenins. J Nat Med. 2017;71:50–58.
- [52]Permanasari AA, Aoki-Utsubo C, et al. An in vitro study of an Artocarpus heterophyllus substance as a hepatitis C antiviral and its combination with current anti-HCV drugs. BMC Complement Med Ther. 2021;21:260.
- [53]Sun R, Zhang Y, et al. Active components of Ligustrum lucidum inhibiting hepatitis C virus replicase activity. Yao Xue Xue Bao. 2013;48:1390–1396.
- [54]Kim S-J, Jang JY, et al. Ginsenoside Rg3 restores hepatitis C virus–induced aberrant mitochondrial dynamics and inhibits virus propagation. Hepatology. 2017;66:758.
- [55]Vijayakumar K, Rengarajan RL, et al. Psidium guajava leaf extracts and their quercetin protect HepG2 cell lines against CCL4 induced cytotoxicity. Indian J Clin Biochem. 2019;34:324–329.
- [56]Tohmé MJ, Giménez MC, et al. Ursolic acid: A novel antiviral compound inhibiting rotavirus infection in vitro. Int J Antimicrob Agents. 2019;54:601–609.
- [57]Yang H, Oh K-H, et al. Ginsenoside-Rb2 and 20(S)-ginsenoside-Rg3 from korean red ginseng prevent rotavirus infection in newborn mice. J Microbiol Biotechnol. 2018;28:391–396.
- [58]Ramírez M-C, Méndez K, et al. In Vitro Evaluation of Anti-Rotaviral Activity and Intestinal Toxicity of a Phytotherapeutic Prototype of Achyrocline bogotensis (Kunth) DC. Viruses. 2022;14:2394.
- [59]James C, Harfouche M, et al. Herpes simplex virus: global infection prevalence and incidence estimates, 2016. Bull World Health Organ. 2020;98:315–329.
- [60]Sadowski LA, Upadhyay R, et al. Current drugs to treat infections with herpes simplex viruses-1 and -2. Viruses. 2021;13:1228.
- [61]Jiang Y-C, Feng H, et al. New strategies against drug resistance to herpes simplex virus. Int J Oral Sci. 2016;8:1–6.
- [62]Garber A, Barnard L, et al. Review of whole plant extracts with activity against herpes simplex viruses in vitro and in vivo. J Evid-Based Integr Med. 2021;26:2515690X20978394.
- [63]Hassan STS, Masarčíková R, et al. Bioactive natural products with anti-herpes simplex virus properties. J Pharm Pharmacol. 2015;67:1325–1336.
- [64]Ogawa K, Nakamura S, et al. Effective search of triterpenes with Anti-HSV-1 activity using a classification model by logistic regression. Front Chem. 2021;9:763794.
- [65]Xu L, Zhong X-L, et al. Medicinal plants and natural compounds against acyclovir-resistant HSV infections. Front Microbiol. 2022;13.
- [66]Krishna BA, Wills MR, Sinclair JH. Advances in the treatment of cytomegalovirus. Br Med Bull. 2019;131:5–17.
- [67]Schulz U, Solidoro P, et al. CMV Immunoglobulins for the treatment of CMV infections in thoracic transplant recipients. Transplantation. 2016;100 Suppl 3:S5-10.
- [68]Lee S, Chung YH, et al. US28, a virally-encoded GPCR as an antiviral target for human cytomegalovirus infection. Biomol Ther. 2017;25:69–79.
- [69]Murugesan A, Manoharan M. Chapter 16 - Dengue Virus. In: Ennaji MM, editor. Emerg. Reemerging Viral Pathog. Academic Press; 2020. p. 281–359.
- [70]Sylvestre G, Gandini M, et al. Preliminary evaluation on the efficiency of the kit Platelia Dengue NS1 Ag-ELISA to detect dengue virus in dried Aedes aegypti: a potential tool to improve dengue surveillance. Parasit Vectors. 2014;7:155.
- [71]Bhatt S, Gething PW, et al. The global distribution and burden of dengue. Nature. 2013;496:504–507.
- [72]Htun TP, Xiong Z, et al. Clinical signs and symptoms associated with WHO severe dengue classification: a systematic review and meta-analysis. Emerg Microbes Infect. 2021;10:1116–1128.
- [73]Panraksa P, Ramphan S, et al. Activity of andrographolide against dengue virus. Antiviral Res. 2017;139:69–78.
- [74]Paemanee A, Hitakarun A, et al. A proteomic analysis of the anti-dengue virus activity of andrographolide. Biomed Pharmacother. 2019;109:322–332.
- [75]Allard P-M, Leyssen P, et al. Antiviral chlorinated daphnane diterpenoid orthoesters from the bark and wood of Trigonostemon cherrieri. Phytochemistry. 2012;84:160–168.
- [76]Sousa FTG, Nunes C, et al. Anti-Zika virus activity of several abietane-type ferruginol analogues. Rev Inst Med Trop São Paulo. 2020;62:e97.
- [77]Newman C, Friedrich TC, et al. Macaque monkeys in Zika virus research: 1947-present. Curr Opin Virol. 2017;25:34–40.
- [78]Musso D, Gubler DJ. Zika Virus. Clin Microbiol Rev. 2016;29:487–524.
- [79]Abreu LS, do Nascimento YM, et al. Tri- and diterpenoids from Stillingia loranthacea as inhibitors of zika virus replication. J Nat Prod. 2019;82:2721–2730.
- [80]Diallo D, Dia I, et al. Chapter 4 - Emergences of Chikungunya and Zika in Africa. In: Higgs S, Vanlandingham DL, Powers AM, editors. Chikungunya Zika Viruses. Academic Press; 2018. p. 87–133.
- [81]Olivon F, Palenzuela H, et al. Antiviral activity of flexibilane and tigliane diterpenoids from Stillingia lineata. J Nat Prod. 2015;78:1119–1128.
- [82]Gupta DK, Kaur P, et al. Anti-Chikungunya viral activities of aplysiatoxin-related compounds from the marine cyanobacterium Trichodesmium erythraeum. Mar Drugs. 2014;12:115–127.
- [83]Cirne-Santos CC, de Souza Barros C, et al. In vitro studies on the inhibition of replication of Zika and Chikungunya viruses by dolastane isolated from seaweed Canistrocarpus cervicornis. Sci Rep. 2020;10:8263.
- [84]Fields M, Marcuzzi A, et al. Mitochondria-targeted antioxidants, an innovative class of antioxidant compounds for neurodegenerative diseases: Perspectives and limitations. Int J Mol Sci. 2023;24:3739.
- [85]Tan M. Norovirus vaccines: Current clinical development and challenges. Pathogens. 2021;10:1641.
- [86]Pan H-J, Nie X-Q, et al. Effects of four kinds of Chinese medicine monomer on growth of PANC-1 xenograft tumor and studying of molecular mechanism. Zhongguo Zhong Yao Za Zhi Zhongguo Zhongyao Zazhi China J Chin Mater Medica. 2013;38:245–248.
- [87]Liao N, Sun L, et al. Antiviral properties of propolis ethanol extract against norovirus and its application in fresh juices. LWT. 2021;152:112169.
- [88]Xu M, Li X, et al. Lupeol alleviates coxsackievirus B3-induced viral myocarditis in mice via downregulating toll-like receptor 4. J Int Med Res. 2020;48:0300060520910908.
- [89]Lv X-J, Li Y, et al. Antiviral triterpenes from the twigs and leaves of Lyonia ovalifolia. J Nat Prod. 2016;79:2824–2837.
- [90]Wang J, Chen X, et al. Glycyrrhizic acid as the antiviral component of Glycyrrhiza uralensis Fisch. against coxsackievirus A16 and enterovirus 71 of hand foot and mouth disease. J Ethnopharmacol. 2013;147:114–121.
- [91]Song J, Yeo S-G, et al. Antiviral activity of hederasaponin B from Hedera helix against Enterovirus 71 subgenotypes C3 and C4a. Biomol Ther. 2014;22:41–46.
- [92]Zhao C, Xu J, et al. Inhibition of human enterovirus 71 replication by pentacyclic triterpenes and their novel synthetic derivatives. Chem Pharm Bull (Tokyo). 2014;62:764–771.
- [93]Nam HH, Ison MG. Respiratory syncytial virus infection in adults. BMJ. 2019;366:l5021.
- [94]Hu Z, Lin J, et al. Overview of viral pneumonia associated with influenza virus, respiratory syncytial virus, and coronavirus, and therapeutics based on natural products of medicinal plants. Front Pharmacol. 2021;12.
- [95]Chen W, Zhang H, et al. A new triterpenoid from the bulbs of Lilium speciosum var. gloriosoides. Chem Nat Compd. 2019;55:289–291.
- [96]Andrei G, Trompet E, et al. Novel therapeutics for Epstein–Barr virus. Molecules. 2019;24:997.
- [97]Orosco F. Recent advances in peptide-based nanovaccines for re-emerging and emerging infectious diseases. J Adv Biotechnol Exp Ther. 2024;7:106.
- [98]Bentz GL, Lowrey AJ, et al. Using glycyrrhizic acid to target sumoylation processes during Epstein-Barr virus latency. PLoS ONE. 2019;14:e0217578.
- [99]Duan E, Wang D, et al. Suppression of porcine reproductive and respiratory syndrome virus proliferation by glycyrrhizin. Antiviral Res. 2015;120:122–125.
- [100]Orosco FL. Current progress in diagnostics, therapeutics, and vaccines for African swine fever virus: Vet Integr Sci. 2023;21:751–781.
- [101]Tong T, Hu H, et al. Glycyrrhizic-acid-based carbon dots with high antiviral activity by multisite inhibition mechanisms. Small. 2020;16:1906206.
- [102]Yu Z, Yi H, et al. Ginsenoside Rg1 suppresses type 2 PRRSV infection via NF-κB signaling pathway in vitro, and provides partial protection against HP-PRRSV in piglet. Viruses. 2019;11:1045.
- [103]Chen Y, Li H, et al. Ursolic acid derivatives are potent inhibitors against porcine reproductive and respiratory syndrome virus. RSC Adv. 2020;10:22783–22796.
- [104]Pascual-Iglesias A, Sanchez CM, et al. Recombinant chimeric transmissible gastroenteritis virus (TGEV)—porcine epidemic diarrhea virus (PEDV) virus provides protection against virulent PEDV. Viruses. 2019;11:682.
- [105]Orosco FL. Immune evasion mechanisms of porcine epidemic diarrhea virus: A comprehensive review: Vet Integr Sci. 2024;22:171–192.
- [106]Wang D, Fang L, Xiao S. Porcine epidemic diarrhea in China. Virus Res. 2016;226:7–13.
- [107]Scott A, McCluskey B, et al. Porcine epidemic diarrhea virus introduction into the United States: Root cause investigation. Prev Vet Med. 2016;123:192–201.
- [108]Raghuvanshi R, Bharate SB. Recent developments in the use of kinase inhibitors for management of viral infections. J Med Chem. 2022;65:893–921.
- [109]Trinh TBN, Le DH, et al. In vitro antiviral activities of ethanol and aqueous extracts of Vietnamese traditional medicinal plants against Porcine Epidemic Diarrhea virus: a coronavirus family member. VirusDisease. 2021;32:797–803.
- [110]Zhang S, Ding G, et al. Functional characterization of the 1-deoxy-d-xylulose 5-phosphate synthase genes in Morus notabilis. Front Plant Sci. 2020;11.
- [111]Kudoh K, Kawano Y, et al. Prerequisite for highly efficient isoprenoid production by cyanobacteria discovered through the over-expression of 1-deoxy-d-xylulose 5-phosphate synthase and carbon allocation analysis. J Biosci Bioeng. 2014;118:20–28.
- [112]Davies FK, Work VH, et al. Engineering limonene and bisabolene production in wild type and a glycogen-deficient mutant of Synechococcus sp. PCC 7002. Front Bioeng Biotechnol. 2014;2.
- [113]Kiyota H, Okuda Y, et al. Engineering of cyanobacteria for the photosynthetic production of limonene from CO2. J Biotechnol. 2014;185:1–7.
- [114]Bentley FK, Zurbriggen A, et al. Heterologous expression of the mevalonic acid pathway in cyanobacteria enhances endogenous carbon partitioning to isoprene. Mol Plant. 2014;7:71–86.